the Creative Commons Attribution 4.0 License.
the Creative Commons Attribution 4.0 License.
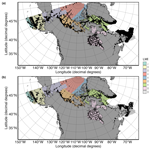
Microbial plankton occurrence database in the North American Arctic region: synthesis of recent diversity of potentially toxic and/or harmful algae
Nicolas Schiffrine
Fatma Dhifallah
Kaven Dionne
Michel Poulin
Sylvie Lessard
André Rochon
Michel Gosselin
The Arctic Ocean is currently undergoing significant transformations due to climate change, leading to profound changes in its microbial plankton communities, including photoautotrophic prokaryotes and eukaryotes (i.e. phytoplankton), as well as hetero-, phago-, and mixotrophic protistan species. Among these unicellular organisms, species of potentially toxic and/or harmful algae (hereafter referred to as HA) are of particular concern, as they pose a threat to human and ecosystem health if they potentially spread into Arctic waters. Despite their importance, the spatiotemporal distribution of these communities in the North American Arctic is poorly understood. To address this gap, we compiled and synthesized a large dataset from various sources, partitioned into nine regions based on the Large Marine Ecosystem classification. Our dataset contains 385 348 georeferenced data points and 18 268 unique sampling events (https://doi.org/10.5281/zenodo.10498858, Schiffrine et al., 2024), encompassing 1442 unique taxa, with Heterokontophyta (notably diatoms) and Dinoflagellata being the most dominant phyla. Our results indicate distinct spatial patterns of diversity, with the highest diversity observed in Atlantic-influenced regions of the North American Arctic. An analysis of the maximum latitude of HA species over time shows a gradual increase, with a notable rise towards the 1990s. However, this trend is likely influenced by increased research at higher latitudes, meaning no substantial spread of HA species into the North American part of the Arctic. Our study underscores the importance of extensive and long-term sampling efforts to understand the Arctic's biodiversity, particularly with respect to documenting the presence and distribution of HA species. While the occurrence of HA species in the Arctic is recognized, our findings highlight the need for further detailed investigations to fully grasp their ecological impacts and variability in the region. Overall, our results provide new insights into the spatial patterns and biodiversity of the microbial plankton communities in the North American Arctic and have implications for understanding the ecological functioning and response of this region to ongoing climate change.
- Article
(3724 KB) - Full-text XML
-
Supplement
(1763 KB) - BibTeX
- EndNote
The Arctic Ocean has become a focal point for climate change research due to its vulnerability to rapid and significant alterations in the environment (Meredith et al., 2019). As a result, the Arctic has been the focus of a growing number of scientific investigations aimed at understanding how these transformations affect the region's ecosystems, people, and global climate. In 2007, the Intergovernmental Panel on Climate Change recognized the Arctic as a region among the most vulnerable to climate change, highlighting the urgent need for further research in this area. Since then, a large body of scientific literature has emerged that explores the effects and implications of climate change on the Arctic marine ecosystem.
The Arctic Ocean is undergoing rapid changes, with surface waters warming nearly 4 times faster than the global average (Rantanen et al., 2022), leading to significant reductions in sea-ice extent and thickness (Hanna et al., 2021; Kacimi and Kwok, 2022). This has resulted in increased melt pond formation (Rösel et al., 2012) and changes in ice dynamics, affecting energy absorption and water column stratification (Carmack et al., 2016), thereby reducing nutrient supply (Yamamoto-Kawai et al., 2009). Additionally, the Arctic Ocean is experiencing some of the fastest rates of ocean acidification (AMAP, 2018), adding to the numerous challenges already faced by this rapidly changing region. These dramatic environmental changes are reshaping the microbial plankton communities, including photoautotrophic prokaryotes and eukaryotes (i.e. phytoplankton), as well as hetero-, phago-, and mixotrophic protistan species, with significant implications for ecosystem structure and function (e.g. Ardyna and Arrigo, 2020). The reduction in sea-ice cover has led to earlier phytoplankton blooms in some regions (Kahru et al., 2011) and has increased open-water periods, potentially leading to a second bloom in autumn (Ardyna et al., 2014). In the Barents Sea, shifts in the current surface velocities have driven poleward intrusions of Gephyrocapsa huxleyi (Lohmann) Reinhardt (previously known as Emiliania huxleyi (Lohmann) Hay & Mohler; Bendif et al., 2019, 2023), a temperate marine calcifying phytoplankton species (Neukermans et al., 2018; Oziel et al., 2020). Conversely, in the less productive waters of the Canada Basin, increased freshwater inflow has led to a transition from nanophytoplankton–diatom communities to picophytoplankton due to altered nutrient availability in the surface layer (Li et al., 2009). The observed changes in physicochemical conditions in the Arctic may also increase the potential risk of proliferation of species of potentially toxic and/or harmful algae (hereafter referred to as HA). Numerous HA species have already been detected in several Arctic regions (Bates et al., 2020; McKenzie et al., 2020). Notably, various toxin-producing diatoms of the genus Pseudo-nitzschia H.Peragallo have been documented in Iceland, Western Greenland, Baffin Bay, Barrow Strait, Beaufort Sea, Bering Strait, and subarctic regions around Norway (Bates et al., 2020; Pućko et al., 2019). Similarly, toxic dinoflagellate species belonging to the genera Alexandrium Halim and Dinophysis Ehrenberg have been detected (Bates et al., 2020; Bruhn et al., 2021; Dhifallah et al., 2021; Okolodkov and Dodge, 1996; Pućko et al., 2019). Olsen et al. (2019) recently documented a red tide of the harmful phototrophic ciliate Mesodinium rubrum (Lohmann) Leegard at the interface between ice and water in newly formed pack ice north of Svalbard during early spring. Their findings suggest that ephemeral blooms of this species are increasingly probable under the context of thinning Arctic sea ice. Nöthig et al. (2015) also described a dominance shift towards the harmful prymnesiophyte Phaeocystis pouchetii (Hariot) Lagerheim, driven by a warm-water anomaly in the Atlantic waters of the West Spitsbergen Current in Fram Strait. Moreover, the increase in maritime traffic due to growing economic and tourism development in the Arctic may elevate the risk of introducing non-native species, including HA species (Chan et al., 2019; Dhifallah et al., 2021). These shifts could have significant implications for the future of Arctic marine ecosystems, impacting the transfer of energy and organic matter through the pelagic food web.
The paucity of data on the diversity and richness of Arctic microbial plankton communities, particularly phytoplankton and other protist species, hinders our ability to fully understand their spatial and temporal variability. Additionally, the complex biogeography of the polar region exacerbates this issue. One way to address these challenges and track potential changes in community structure, dynamics, and phenology is through the use of long-term datasets. The emergence of digital archives of biological data, such as the Global Biodiversity Information Facility (GBIF; https://www.gbif.org/, last access: 27 August 2024) and the Ocean Biogeographic Information System (OBIS; https://www.obis.org/, last access: 27 August 2024), has enabled the identification of significant patterns in the global distribution of microbial plankton diversity as well as the occurrence, toxicity, and associated risks posed by HA species (Hallegraeff et al., 2021; Righetti et al., 2019). Despite numerous studies that have utilized long-term datasets to monitor changes in Arctic microbial plankton diversity and dominance, most of these studies have been conducted in specific regions of the Arctic (Blais et al., 2017; Freyria et al., 2021). Furthermore, previous reports on the diversity of Arctic microbial plankton communities have not included essential information, such as geographic coordinates and dates, limiting the ability to assess potential changes in diversity and dominance (e.g. Poulin et al., 2011). To date, there has been no effort to comprehensively combine data from various sources, such as OBIS, GBIF, and both published and unpublished datasets, into a unified database specifically for the North American Arctic. This study aims to fill this gap by creating an extensive database on a pan-American Arctic scale. This database will facilitate the investigation of global trends in the biogeography, diversity, and composition of microbial plankton taxa across the North American region of the Arctic Ocean, thereby addressing the limitations of existing quantitative data.
2.1 Data acquisition
Our database consists of microbial plankton occurrences (i.e. presences and abundances greater than zero), including photoautotrophic prokaryotes and eukaryotes (i.e. phytoplankton), as well as hetero-, phago-, and mixotrophic protistan species. These data were compiled from web-based search engines and queries in online databases, such as OBIS (https://obis.org, last access: 20 November 2020), GBIF (https://www.gbif.org, last access: 16 November 2020), and PANGAEA (https://www.pangaea.de/, last access: November 2020). Occurrence data from OBIS (n=575 200) and GBIF (n=197 439) were first downloaded using the keywords “Chromista” and “Plantae”; from 45 to 90° N and from 40 to 180° W, without temporal restriction. Occurrence data from PANGAEA (n=1994) were collected using the keywords: “Chromista”, “Phytoplankton”, “Taxonomy”, “Harmful algal bloom”, “Arctic Ocean”, “Polar”, and several combinations of these keywords. We supplemented the data with records from ArcticNet campaigns (n=43 982) and individual studies (n=90 479). We included the “sourceArchive” column (Table 1) to specify the origin of each record (i.e. GBIF, OBIS, ArcticNet, or individual studies). We standardized the column names to ensure compatibility between different datasets and to adhere to the Darwin Core standard (https://dwc.tdwg.org/, last access: 27 August 2024), resulting in a comprehensive dataset of 909 094 data points (Schiffrine et al., 2024).
2.2 Biogeographic classification
Our global database was divided into hexagonal bins using the R package dggridR (version 3.1.0; https://github.com/r-barnes/dggridR, last access: 27 August 2024; Barnes and Sahr, 2020), with a resolution of 2591.40183 km2. The chosen grid resolution strikes a balance between providing sufficient spatial resolution to capture ecological patterns and minimizing computational requirements. Each grid cell was then assigned a corresponding Large Marine Ecosystem (LME) region using the spatial polygons obtained from the “mr_shp” function of the R package mregions (version 0.1.9; Chamberlain et al., 2024). Arctic LMEs are defined by ecological criteria, including bathymetry, hydrography, productivity, and trophically linked populations, and are integral to ecosystem-based management approaches with a five-module framework focused on productivity, fish and fisheries, pollution and ecosystem health, socioeconomics, and governance (PAME, 2013). Conserving only grid cells labelled as “arctic” according to the LME classification (PAME, 2013), this new dataset contains 4458 grid cells partitioned into nine different regions and 550 033 data points (Schiffrine et al., 2024).
2.3 Data quality control
Each record underwent a verification process to ensure the accuracy of taxonomic identification. First, we used the AlgaeBase database, and the application programming interface (API) key provided by the AlgaeBase team to validate each record as an accepted name (http://www.algaebase.org/, last access: 27 August 2024; AlgaeBase, 2023). If a record was not validated through this process, we performed a secondary verification with the “wm_records” function from the R package worrms (version 0.4.3; Chamberlain and Vanhoorne, 2023), using the World Register of Marine Species database (WoRMS; http://www.marinespecies.org, last access: 27 August 2024; Ahyong et al., 2023). If the taxonomic identification could not be found in either of these databases, we assigned the record to the next higher taxonomic classification level (n=39). These modifications were specified in the “ReduceName” column (Table 1). In order to maintain data quality and avoid loss of information, we manually adjusted a total of 249 taxonomic names, with the modified names indicated in the “ModifiedName” column (Table 1). Taxonomic records that included qualifiers such as “aff.” (n=40) and “cf.” (n=95) were categorized at the species level in our dataset to simplify taxonomic classification. While this simplification enhances dataset accessibility, it is crucial to acknowledge the potential introduction of errors due to a certain degree of uncertainty associated with species identification. To maintain transparency, qualifiers originally denoted by cf. and aff. were thoughtfully preserved in the “openNomenclature” column (Table 1). This approach strikes a balance between simplification and taxonomic rigour, enabling users to recognize the initial uncertainty in identification and facilitating further investigation or refinement of taxonomic assignments as necessary. Taxonomic records with qualifiers, such as “sp.” (n=193) or “spp.” (n=324), and those indicating a “group” (e.g. Pseudo-nitzschia seriata group; n=27), “complex” (e.g. Gymnodinium/Gyrodinium complex; n=3), or containing multiple species names (e.g. Pseudo-nitzschia delicatissima/Pseudo-nitzschia pseudodelicatissima; n=12) were categorized at the genus level in the dataset. These qualifiers are denoted in the openNomenclature column (Table 1). Less than 1 % of the records in our dataset could not be identified in either the AlgaeBase or WoRMS databases. The original taxonomic names were retained in the “verbatimScientificName” (Table 1), allowing for traceability to the harmonized names.
Table 1Detailed description of the columns. For further details, see https://dwc.tdwg.org/ (last access: 27 August 2024).
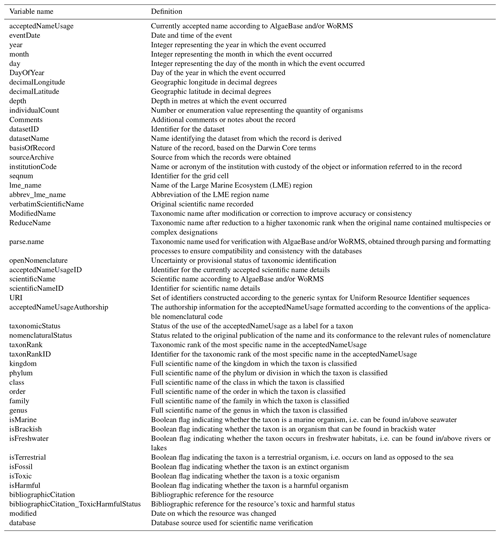
2.4 List of potentially toxic and/or harmful algal species
In the context of this study, “HA” is used as an abbreviation to refer collectively to potentially toxic and/or harmful algal species. Our comprehensive global list of HA species consists of the IOC-UNESCO taxonomic reference list (http://www.marinespecies.org/hab/; last access: 20 June 2023; Lundholm et al., 2009). We subsequently supplemented this list by incorporating the taxa list of Bates et al. (2020, 2019), which notably included the Ciliophora Mesodinium rubrum (Lohmann) Leegard. We chose to retain M. rubrum due to its significant ecological implications (McKenzie et al., 2020; Olsen et al., 2019). We excluded the dinoflagellate Protoperidinium crassipes (Kofoid) Balech from Bates et al. (2020), as this heterotrophic species appears to act more as a toxin vector than a toxin producer (e.g. Tillmann et al., 2009). Each taxon was verified as described in the previous section and merged based on the “acceptedNameUsage” column. We assigned the toxic and/or harmful status to each record, following the criteria of Lundholm et al. (2009) and Bates et al. (2020, 2019). This compiled list includes 113 Dinoflagellata, 49 Heterokontophyta, 43 Cyanobacteriota, 11 Haptophyta, and one Ciliophora species. Of these, 205 species have been identified as toxic (indicated by a flag in the “isToxic” column; Table 1), 7 are considered harmful (indicated by a flag in the “isHarmful” column; Table 1), and 5 species remain under debate regarding their toxic and/or harmful status (flagged in both isToxic and isHarmful columns; Table 1).
2.5 Data merger and synthesis
The filters implemented during the data merging and synthesis process aimed to ensure the quality and relevance of the dataset. The filters applied were as follows:
-
Records without year information were removed to ensure data quality and enable meaningful temporal analysis, as the absence of this crucial temporal component would limit the dataset's usability for studying time-dependent patterns or trends.
-
Records with depths greater than 2500 m were excluded, considering the specific characteristics and depth ranges of the Arctic region based on bathymetry data.
-
Records classified as “fossil only” or “fossil” in either the AlgaeBase or WoRMS databases (e.g. “isFossil” column; Table 1) were excluded to focus only on currently occurring microbial plankton species. However, records classified as freshwater or brackish according to the AlgaeBase or WoRMS databases (i.e. “isFreshwater” and “isBrackish” columns; Table 1) were retained to account for their ecological relevance and potential responses to changing Arctic conditions, given the Arctic's connection to freshwater and brackish coastal regions.
-
Records not found in either AlgaeBase or WoRMS were excluded to ensure the inclusion of taxonomically validated and accepted names.
-
Taxa belonging to specific kingdoms (i.e. Animalia, Fungi, and Acritarcha), phyla (i.e. Foraminifera, Oomycota, Rhodophyta, and Retaria), and classes (i.e. Phaeophyceae and Ulvophyceae) were excluded to maintain the focus on microbial plankton species.
-
Records identified at a taxonomic level higher than genus were removed from the dataset to ensure consistent and accurate taxonomic classification at the genus level. Retaining records at the genus level allows for a more detailed understanding of the composition of microbial plankton communities in the study area.
-
Duplicate records were removed, using the following columns: “day”, “month”, “year”, “depth”, “decimalLatitude”, “decimalLongitude”, “verbatimScientificName”, “scientificName”, “acceptedNameUsage”, “basisOfRecord”, and “individualCount” (Table 1). This step ensured that each unique sampling event was represented by a single record in the dataset.
After applying these filters, the dataset contains 385 348 individual georeferenced data points and 18 268 unique sampling events (Fig. 1a; Schiffrine et al., 2024). To access the comprehensive diversity of HA species, we further subset the database based on the isToxic and isHarmful columns (Table 1), resulting in a dataset with a total of 48 555 georeferenced data points of HA species and 6744 unique sampling events (Fig. 1b; Schiffrine et al., 2024).
2.6 Data analysis
The size of each LME region was determined by calculating the total number of grid cells (i.e. ntotal). For each LME region and each month, the number of grid cells containing records was counted and summed per year (i.e. nsampled). This value was then divided by ntotal to estimate the percentage of the region that was sampled (or sample coverage) that specific year within each LME region. The same method was applied to the HA data subset, where nHA sampled represents the number of grid cells containing HA records summed per year. Mapping and statistical analysis were performed on the filtered dataset (see Sect. 2.6) in R (R version 4.4.1; R Core Team, 2024), using the tidyverse (version 2.0.0; Wickham et al., 2019), ggOceanMaps (version 2.2.0; https://mikkovihtakari.github.io/ggOceanMaps/, last access: 27 August 2024; Vihtakari, 2021), vegan (version 2.6-6.1; Oksanen et al., 2024), and betapart (version 1.6; Baselga and Orme, 2012) packages.
3.1 Spatiotemporal coverage
The use of long-term datasets has significantly improved our understanding of (1) microbial plankton taxa distribution and diversity and (2) the underlying drivers of these patterns at both local (McKenzie et al., 2020; Nohe et al., 2020) and global scales (Benedetti et al., 2021; Hallegraeff et al., 2021; Righetti et al., 2019). However, currently available databases on microbial plankton occurrences only provide limited information on the Arctic Ocean. Although PhytoBase (i.e. Righetti et al., 2020) is one of the most comprehensive and up-to-date sources of information on phytoplankton occurrence, data above 60° N are generally underrepresented. Furthermore, the recent study by Hallegraeff et al. (2021) did not specifically address the evolution of HA blooms in the Arctic Ocean; instead, they grouped the North American region of the Arctic Ocean within the broader region of “East Coast America”.
Despite the existence of several published microbial plankton taxa lists specifically focused on the North American sector of the Arctic Ocean, there is currently a lack of a comprehensive and freely available standardized database accessible to the scientific community. To fill this gap, our project aimed to compile and integrate a large and diverse collection of data from multiple sources. The objective was to create a comprehensive database covering the distribution of microbial plankton, including photoautotrophic prokaryotes and eukaryotes (i.e. phytoplankton), as well as hetero-, phago-, and mixotrophic protistan species with a particular focus on HA species across the North American sector of the Arctic Ocean. Our efforts greatly expanded the spatiotemporal coverage of microbial plankton data across all LME regions in this sector of the Arctic Ocean compared with PhytoBase. Our database covers an impressive time span of 132 years, from 1888 to 2020, with 95 % of the data collected after 1963. Sampling was mainly concentrated between the months of June and September, corresponding to reduced ice cover in the Arctic Ocean and better ship accessibility to the Arctic. The spatial distribution of the records was highly unbalanced, with 82 % of data records falling in the Labrador–Newfoundland region alone, followed by the Canadian Eastern Arctic–West Greenland region with 8 % (Fig. S1). The remaining areas contribute smaller proportions, ranging from 0.1 % to 5 % of the data records (Fig. S1). The dataset spans a depth range from 0 to 1010 m. However, 17 % of the dataset lacks depth information, necessitating caution when interpreting the vertical distribution of phytoplankton and other protists. This is particularly significant in the Arctic marine environment, where subsurface chlorophyll-a maxima are common (e.g. Martin et al., 2012). The scarcity of depth data may lead to an underestimation of biodiversity in these deeper layers. While 83 % of the entries include depth information, allowing for some general statements regarding vertical distribution, the vast majority of data focuses on surface layers (95 %), and the gaps in depth records impose certain limitations on our ecological interpretations. Regarding the types of records within the dataset (i.e. basisOfRecord column; Table 1), the majority (71 %) are categorized under “HumanObservation”, which includes occurrences documented through field notes, literature, or records without physical or machine-recorded evidence. Another significant portion, 19 % of the dataset falls under the “PreservedSpecimen” category, representing samples that have been treated with fixatives for preservation.
3.2 Taxonomic coverage
A total of 1442 unique taxa were recorded in our study. This number falls within the range reported by Archambault et al. (2010) and Poulin et al. (2011) for the same region (i.e. 1657 and 1229 taxa, respectively). It is essential to acknowledge that both Archambault et al. (2010) and Poulin et al. (2011) conducted their analyses based on literature reviews predominantly reliant on microscopic observations. In more recent comprehensive pan-Arctic taxonomic inventories using genomic techniques, Lovejoy et al. (2017) and Ibarbalz et al. (2023) reported 2241 and 3082 different operational taxonomic unit (OTU) taxa, respectively. The discrepancy in reported taxa between our study and the aforementioned studies can be attributed to the fundamental differences in our respective approaches – our reliance on mainly microscopic observations (i.e. >90 %) versus their exclusive use of genomic data. Genomic techniques possess the capacity to identify a broader spectrum of species, including those of smaller size or those less conspicuous under microscopic examination, such as the Mamiellophyceae Micromonas polaris Simon, Foulon & Marin. Microscopic observations, which constitute a substantial portion of our dataset, inherently introduce certain biases. They may overlook rare or small species (<3 µm) and encounter challenges related to precise species identification, compounded by considerations such as the choice of fixative (e.g. acidic Lugol's solution or formalin; Sournia, 1978). As a result, our study may not offer a fully comprehensive representation of total species richness, particularly concerning rare or molecularly detectable taxa.
In this study, Heterokontophyta and Dinoflagellata were the most commonly occurring phyla, accounting for approximately 45 % and 36 % of total occurrences, respectively (Fig. 2a). Within the phylum Heterokontophyta, which notably included diatoms (Guiry et al., 2023), the genus Chaetoceros Ehrenberg was the most frequently observed, followed by Thalassiosira Cleve, accounting for 24 % and 14 % of total Heterokontophyta occurrences, respectively (Fig. 2b). Tripos Bory and Gyrodinium Kofoid & Swezy were the two most abundant genera in the phylum Dinoflagellata, accounting for 20 % and 16 % of total Dinoflagellata occurrences, respectively (Fig. 2c). The observed predominance of Heterokontophyta in this study, particularly the genera Chaetoceros and Thalassiosira, is in line with the general understanding of Arctic phytoplankton and other protist diversity (Lovejoy et al., 2017; Poulin et al., 2011). On the other hand, the findings for the phylum Dinoflagellata contrast with prior research that has highlighted the predominance of the genus Protoperidinium Bergh (Okolodkov and Dodge, 1996). The exceptionally high occurrence of Tripos and Gyrodinium should be interpreted with caution. These two genera are mainly observed in the Labrador–Newfoundland region, where the majority of the data collected originates from the Continuous Plankton Recorder (CPR; Fig. S2). It is important to note that the CPR uses a large mesh size (270 µm) (e.g. Richardson et al., 2006), resulting in an overrepresentation of larger taxa, such as Tripos and Gyrodinium.
Cyanobacteria are notably scarce in Arctic waters, and their ecological roles appear to be taken over by picoeukaryotic algae (Buitenhuis et al., 2012; Pedrós-Alió et al., 2015). Studies indicate a marked decrease in the cell abundance of oceanic picocyanobacteria with increasing latitude in the Northern and Southern hemispheres. Among these, Prochlorococcus Chisholm, Frankel, Goericke, Olson, Palenik, Waterbury, West-Johnsrud & Zettler ex Komárek et al., the most abundant photosynthetic genus in tropical oceans, is notably absent from polar waters (Flombaum et al., 2013). The International Census of Marine Microbes (ICoMM) surveys retrieved fewer than 30 true Cyanobacterial tags overall, reflecting their scarcity in Arctic marine waters (Lovejoy et al., 2011). In addition, a study of molecular diversity in the Beaufort Sea identified picocyanobacteria that were mostly affiliated with freshwater and brackish Synechococcus Nägeli lineages, rather than oceanic ones (Waleron et al., 2007). Similarly, a 16S rRNA gene study of bacterial communities in the Beaufort Sea and Amundsen Gulf found that cyanobacteria were not among the top 50 most abundant bacterial taxa (Comeau et al., 2011). Our study revealed the presence of 26 distinct cyanobacteria entries, with 12 identified at the genus level and 14 at the species level. These 26 taxa encompass a diverse range of Cyanobacteria, with Synechococcus being the most frequently detected genus, accounting for 91 % of the cyanobacterial occurrences. Overall, cyanobacterial occurrences represent a small fraction of the total (0.8 %). These results are consistent with the hypothesis of an allochthonous origin of Cyanobacteria in the coastal Arctic Ocean, as all species observed in our study are labelled by AlgaeBase as freshwater species (Schiffrine et al., 2024). Our findings also support previous studies suggesting that the presence of Cyanobacteria in the Arctic may be underestimated and highlight the need for further research to understand their ecological significance.
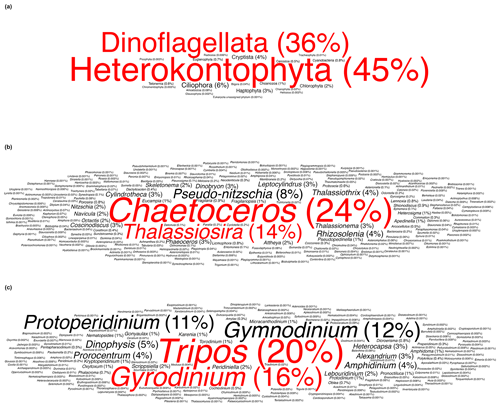
Figure 2Word cloud representation of the (a) most commonly occurring phyla, (b) most commonly occurring genera within the phylum Heterokontophyta, and (c) most commonly occurring genera within the phylum Dinoflagellata. Words are sized proportionally to their occurrence and coloured to distinguish the two most frequent categories.
A well-established concept is the latitudinal gradient of diversity, where the highest levels of diversity are typically found near the Equator, gradually diminishing towards the poles (Ibarbalz et al., 2019; Righetti et al., 2019). However, this pattern is not universally consistent across all taxa. Chaudhary et al. (2016) have highlighted that diversity may exhibit a bimodal distribution for certain groups, with peaks occurring in the mid-latitudes rather than at the Equator and a notable decline in species richness within equatorial regions. Furthermore, patterns of phytoplankton diversity exhibit significant variation between different Longhurst provinces (Hörstmann et al., 2024). The primary driver behind these patterns is likely ocean temperature variability (Chaudhary et al., 2017; Ibarbalz et al., 2019). While environmental conditions undoubtedly contribute to these diversity patterns, the scarcity of data may also account for the observed low diversity. Righetti et al. (2020) reported a total of 1704 phytoplankton species, including 239 species within the same grid used in our study. However, our study detected an additional 1359 taxa, of which 532 belonged to Heterokontophyta and 363 to Dinoflagellata. Our results indicate that previous research may have significantly underestimated the biodiversity of Arctic phytoplankton and other protists (Righetti et al., 2019). Such underestimation may bias our understanding of the latitudinal gradient of diversity.
3.3 Difference in species richness according to the Arctic LME regions
LME regions show substantial variation in the nature of the data (i.e. basisOfRecord column; Table 1; Fig. S3a) and data provenance (i.e. sourceArchive column; Table 1; Fig. S3a), resulting in high variation in sampling coverage (Fig. S4). Regions with higher contributions from OBIS/GBIF, such as the Labrador–Newfoundland region (Fig. S3a), tend to have more extensive datasets. This leads to greater sampling coverage (Fig. S4) and a higher probability of capturing a wider range of species, providing a more comprehensive representation of local biodiversity. In contrast, regions with higher contributions from individual studies, such as the Aleutian Islands, Central Arctic, or Canadian High Arctic–North Greenland regions (Fig. S3a), may have been the focus of more specific scientific research. This results in lower sampling coverage (Fig. S4) and may underrepresent species richness, potentially leading to an incomplete understanding of the true species composition in these areas. To address this concern, we employed the Chao2 index, a widely used nonparametric method for estimating species richness in a community (Chao and Shen, 2003). The Chao2 index is particularly valuable as it accounts for rare species, providing a more accurate estimate of species richness in datasets with uneven sampling effort. The application of the Chao2 index allows us to assess α-diversity, i.e. diversity on a local scale, within each LME region, especially when working with frequency counts or presence/absence data. The Chao2 index reveals significant differences in diversity among the LME regions (Fig. 3). The Canadian Eastern Arctic–West Greenland and Beaufort Sea regions exhibited the highest Chao2 index values, indicating higher species richness and diversity within their phytoplankton and other protist communities. In contrast, the Aleutian Islands and Central Arctic regions had the lowest values, suggesting lower species richness and diversity in these areas. The East Bering Sea, Hudson Bay Complex, Labrador–Newfoundland, and Northern Bering–Chukchi Seas regions also showed moderate to high Chao2 index values, indicating varying levels of species richness and diversity across these regions.
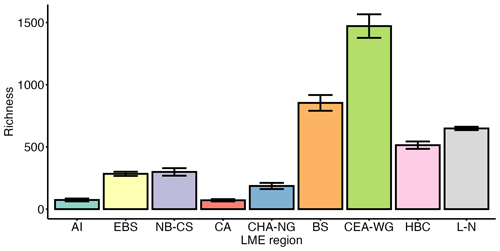
Figure 3Chao2 index for each Large Marine Ecosystem (LME) region. Error bars represent the standard error (i.e. SE). LME regions are labelled as follows: AI (Aleutian Islands), n=28; EBS (East Bering Sea), n=401; NB-CS (Northern Bering–Chukchi Seas), n=122; BS (Beaufort Sea), n=318; CA (Central Arctic), n=29; CHA-NG (Canadian High Arctic–North Greenland), n=19; CEA-WG (Canadian Eastern Arctic–West Greenland), n=656; HBC (Hudson Bay Complex), n=177; L-N (Labrador–Newfoundland), n=7268.
To further analyse local diversity, we used species accumulation curves (SACs) to illustrate the number of species sampled relative to the level of sampling effort (Thompson and Withers, 2003). SACs typically reach an asymptote when sufficient sampling effort is achieved, enabling us to estimate the comprehensiveness of species richness detection. In our analysis, we computed SACs based on the number of species observed in each grid cell for each month of every year, yielding valuable insights into species richness and the extent of saturation (i.e. completeness of species richness detection) between regions (Fig. 4). The Hudson Bay Complex and Labrador–Newfoundland regions exhibited saturation at cumulative richness levels of around 400 and 600 taxa, respectively (Fig. 4), indicating that a significant proportion of the taxa present in these regions had been sampled. Conversely, the SACs for other regions did not reach a plateau, suggesting that the sampling effort was insufficient to capture the complete diversity (Fig. 4). This pattern was particularly pronounced in the northernmost regions, such as the Central Arctic and Canadian High Arctic–North Greenland (Fig. 4).
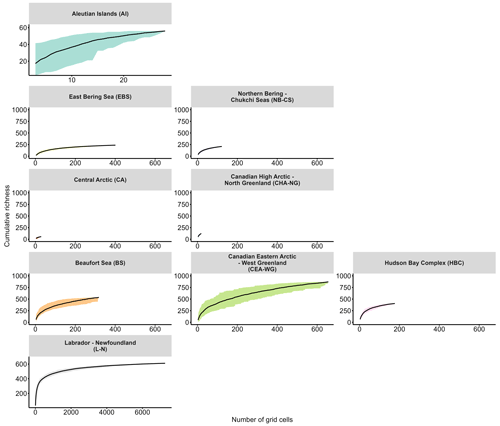
Figure 4Cumulative richness and standard deviation (i.e. SD; coloured area) as a function of the number of grid cells for each month of every year for each Large Marine Ecosystem (LME) region. LME region abbreviations are given in parentheses.
The Central Arctic region, known for its extreme environmental conditions, such as low nutrient concentrations and prolonged annual sea-ice cover (Codispoti et al., 2013), exhibits a lower Chao2 index compared with other regions (Fig. 3). However, the SAC did not saturate (Fig. 4), indicating that the actual diversity in this region may be higher than observed in this study. Despite the inflow of nutrient-rich water through the Bering Strait (Torres-Valdés et al., 2013), which contributes to the high productivity in Pacific regions, such as the East Bering Sea and Northern Bering–Chukchi Seas (Tremblay et al., 2015), these regions display a relatively low Chao2 index (Fig. 3). One possible explanation for this observation is that the sampling effort conducted in these regions may not have been sufficient to capture the complete range of species diversity, leading to an underestimation of richness. This is supported by the SACs (Fig. 4), which show that the curves for both regions do not reach a plateau, indicating that the sampling effort was insufficient to fully assess the diversity present in these areas. The Labrador–Newfoundland region displays an intermediate Chao2 index (Fig. 3), although the SAC indicates that the majority of species have been recorded (Fig. 4). This saturation can be attributed to the provenance (i.e. sourceArchive column; Table 1; Fig. S3a) and the nature (i.e. basisOfRecord column; Table 1; Fig. S3b) of the data. Notably, 90 % of the data come from OBIS, the majority of which were collected using the Continuous Plankton Recorder (CPR). The CPR's sampling methods, which involve a large mesh size (270 µm), fixed sampling depth (5–10 m), and high sampling speed (15–20 kn), are effective at capturing larger and more robust species but tend to miss smaller and more delicate species, as well as those that are not consistently present in the surface mixed layer (Richardson et al., 2006). As a result, the intermediate Chao2 index and the apparent saturation in the SACs may coexist. This is because the limitations of the CPR result in undersampling of certain species, leading to apparent saturation in the species accumulation curves, while the Chao2 index may suggest that more species are present but not captured by the current sampling methods. The Chao2 index values for both the Beaufort Sea and the Canadian Eastern Arctic–West Greenland regions were remarkably high (Fig. 3). This is consistent with previous observations of relatively high diversity in the Canadian Eastern Arctic–West Greenland region (Joli et al., 2018; Kalenitchenko et al., 2019). However, it comes as a surprising result for the Beaufort Sea region, where (except during episodic upwelling events) the water column is highly stratified and nutrient concentrations in the surface mixed layer are extremely low, especially in the northern part (Ardyna et al., 2017). This typically leads to a community with relatively low diversity and a high prevalence of picoeukaryotes, mostly represented by the psychrophilic prasinophyte Micromonas polaris (Balzano et al., 2012; Coupel et al., 2015; Tremblay et al., 2009). The relatively high Chao2 index value for the Beaufort Sea region may be explained by the fact that most of the samples were collected from nearshore areas (Fig. S5). These nearshore areas are known for their high productivity (Ardyna et al., 2017), likely due to their exposure to nutrient-rich waters that can support the growth and diversity of microbial plankton communities. Another explanation could be the influence of a stable surface mixed layer in the Beaufort Gyre that provides multiple ecological niches, further contributing to the observed high diversity. Nevertheless, the SACs for both regions indicate that sampling efforts in these areas are incomplete (Fig. 4). This implies that diversity may be underestimated and underscores the importance of further sampling to gain a more accurate understanding of local biodiversity in both the Beaufort Sea and the Canadian Eastern Arctic–West Greenland regions.
The β-diversity assessment provides valuable insights into the dissimilarity of the taxa composition between multiple samples, enabling researchers to understand the variation in biodiversity across different spatial scales (Whittaker, 1972). In this study, we used the Sørensen dissimilarity index (βSØR) as the β-diversity index to determine the proportion of taxa that are not shared between LME regions. The βSØR values range from 0 to 1, where 0 indicates identical taxonomic composition at all sites, and 1 represents completely different sets of taxa (Baselga, 2010). Our analysis revealed the subdivision of the LME regions into three distinct clusters based on their taxa composition (Fig. 5). The first cluster, known as the “Pacific cluster”, includes the Aleutian Islands, East Bering Sea, and Northern Bering–Chukchi Seas regions. The second cluster, referred to as the “Northern Arctic cluster”, encompasses the Central Arctic and Canadian High Arctic–North Greenland regions. Lastly, the third cluster, named the “Mixed Arctic cluster”, consists of the Beaufort Sea, Canadian Eastern Arctic–West Greenland, Hudson Bay Complex, and Labrador–Newfoundland regions. The grouping of the Aleutian Islands, East Bering Sea, and Northern Bering–Chukchi Seas regions is anticipated owing to their common water supply and circulation patterns, which involve receiving water inflows from the Pacific Ocean through the Bering Strait (Rudels and Carmack, 2022). Consequently, this leads to comparable environmental conditions and nutrient inputs, which, in turn, explain the observed similarities in the composition of microbial plankton communities. Similarly, the Central Arctic and Canadian High Arctic–North Greenland regions share a common water circulation pattern in the Arctic Ocean (Rudels and Carmack, 2022). The common circulation pattern, combined with similar environmental characteristics, contributes to the similarities in taxonomic composition between these two regions. The inclusion of the Beaufort Sea region with Atlantic-dominant regions (i.e. Canadian Eastern Arctic–West Greenland, Hudson Bay Complex, and Labrador–Newfoundland) into one unique cluster may initially seem contradictory due to its geographic location outside the Atlantic side of the Arctic and lack of direct influence from Atlantic waters (Rudels and Carmack, 2022). However, this clustering is based on similarities in taxa composition rather than geographic proximity or environmental conditions. Despite its location, the Beaufort Sea region exhibits a higher resemblance in taxa composition to the Canadian Eastern Arctic–West Greenland, Hudson Bay Complex, and Labrador–Newfoundland regions compared with other regions in the dataset. This unexpected similarity may be attributed to oceanic circulation patterns and water mass transport mechanisms that connect these regions (Rudels and Carmack, 2022). These circulation patterns and transport mechanisms may facilitate the dispersal of taxa from the Beaufort Sea to Atlantic-dominated regions, thereby influencing the observed similarities in taxa composition (Wassmann et al., 2015). Such circulation-driven dispersal was documented by Reid et al. (2007), who observed the spread of the diatom Neodenticula seminae (Simonsen & T.Kanaya) Akiba & Yanagisawa from the northwestern Arctic to the Atlantic side, possibly through the Canadian Arctic Archipelago and/or Fram Strait. This observation provides additional support for the concept of shared microbial plankton composition influenced by oceanic circulation. These findings provide important insights into the biogeographic patterns of microbial plankton communities in the Arctic LME regions and highlight the importance of considering both geographic and ecological factors when interpreting these patterns.
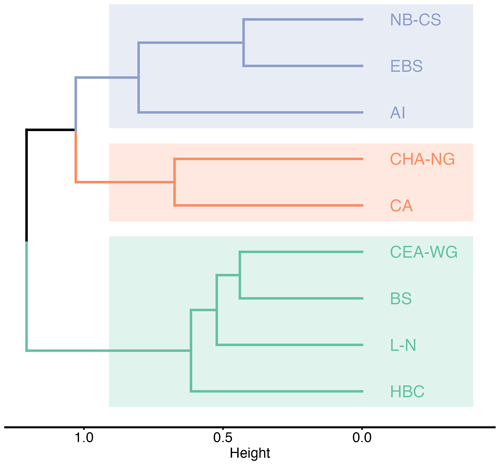
Figure 5Cluster analysis of the β-diversity Sørensen dissimilarity index (βSØR) between the different Large Marine Ecosystem (LME) regions obtained with Ward's cluster method. LME regions are labelled as follows: NB-CS (Northern Bering–Chukchi Seas), EBS (East Bering Sea), AI (Aleutian Islands), CHA-NG (Canadian High Arctic–North Greenland), CA (Central Arctic), CEA-WG (Canadian Eastern Arctic–West Greenland), BS (Beaufort Sea), L-N (Labrador–Newfoundland), and HBC (Hudson Bay Complex).
3.4 Diversity of potentially toxic and/or harmful algal species
The presence of HA species has been a well-known concern in temperate marine and freshwater ecosystems, but their occurrence in the Arctic marine ecosystem is relatively new. With the ongoing climate change in the Arctic Ocean, there is a high probability that the frequency of HA occurrence will increase, notably by stimulating cyst germination (Anderson et al., 2021). Furthermore, the expansion of HA distributions from other regions due to increased ship traffic in the Arctic may further exacerbate this problem (e.g. Chan et al., 2019). HA species pose substantial risks to both human and ecosystem health, and they can cause massive economic losses via fish kills. The phycotoxins produced by some of these organisms can bioaccumulate in higher-trophic-level organisms, including molluscs, seabirds, and marine mammals. When transferred to higher trophic levels, these phycotoxins can result in massive mortality or in neurological or gastrointestinal adverse effects if consumed at concentrations that surpass safe thresholds. In the Alaskan sector of the Bering Sea, concentrations of these toxins have been detected in shellfish tissues that could pose a health risk to local populations (Gao et al., 2019). This issue is particularly important, as northern populations rely on traditional harvesting of fish, shellfish, and marine mammals for subsistence food.
Of the 217 HA species compiled from Lundholm et al. (2009) and Bates et al. (2020, 2019) (see Sect. 2.4 for details; Schiffrine et al., 2024), our database identified 59 species. Notably, our study detected a higher number of HA species compared with previous studies conducted by Poulin et al. (2011) and Pućko et al. (2019), who reported 36 and 27 species, respectively, after updating their species lists with revised taxonomy. It is noteworthy that both studies primarily aggregated data from literature reviews based on microscopic observations. Our study contributed an additional 25 species, including 16 species from the phylum Dinoflagellata and 7 species from the phylum Heterokontophyta. It is important to note that at least 11 species reported by Poulin et al. (2011) were not detected in the present work, as they occur in other Arctic regions not covered in our study, such as Alexandrium minutum Halim observed in the Russian and Scandinavian regions.
Many of the species highlighted in our study are of particular concern for the Arctic Ocean due to their production of phycotoxins. Based on Lundholm et al. (2009) and Bates et al. (2020, 2019), we identified 48 potentially toxin-producing species, as indicated by the isToxic flag (Table 1). Of the 73 accepted species included in the genus Pseudo-nitzschia (AlgaeBase, 2023), 28 are known to produce domoic acid (Bates et al., 2019; Lundholm et al., 2009), with 9 of these toxin-producing species being present in our database (Schiffrine et al., 2024). Meanwhile, at least 16 of the 45 accepted species in the genus Alexandrium are known to be toxic (AlgaeBase, 2023; Lundholm et al., 2009), but only 5 species have been recorded in our database (Schiffrine et al., 2024). The dinoflagellate genus Dinophysis has 276 phototrophic and heterotrophic accepted species worldwide (AlgaeBase, 2023), and 10 of these species have been found to produce various toxins (Lundholm et al., 2009). Additionally, 14 out of the 133 accepted species of the genus Prorocentrum Ehrenberg have been confirmed to produce a range of toxins (AlgaeBase, 2023; Lundholm et al., 2009). Our database contains at least six and five species from the genera Dinophysis and Prorocentrum, respectively (Schiffrine et al., 2024).
Surprisingly, we observed the presence of Pyrodinium bahamense Plate in our dataset, an occurrence published by MGnify (https://www.ebi.ac.uk/metagenomics, last access: 27 August 2024; e.g. Mitchell et al., 2020) and hosted on GBIF (https://www.gbif.org/dataset/b42d7c7f-43e5-4e24-abd7-fab3b4fceb09, last access: 27 August 2024). This observation is intriguing, as P. bahamense is typically associated with warm tropical waters (Morquecho, 2019), and its presence in Arctic waters is unexpected. The publication referenced by MGnify (e.g. Joli et al., 2018) does not mention the presence of P. bahamense, a fact also confirmed by Nathalie Joli, Connie Lovejoy, and Michel Gosselin (personal communication, 2022), further adding to the uncertainty of this occurrence. This discrepancy suggests potential misidentifications when using data from platforms such as MGnify, which are primarily designed for microbiome analysis and may not always accurately identify species. Similarly, we observed the presence of the Pelagophyceae Aureococcus anophagefferens Hargraves & Sieburth, in the Canadian Eastern Arctic–West Greenland region. Unlike the occurrence of P. bahamense, this finding was published by Elferink et al. (2017b) as a supplement to their work (e.g. Elferink et al., 2017a). Therefore, this occurrence has undergone a peer-review process, reducing the likelihood of misidentification and increasing the credibility of the occurrence. Additionally, the presence of this species in the Arctic region was also reported by Ibarbalz et al. (2023), further supporting its presence in this region. Nevertheless, retaining such data is standard practice in scientific research to ensure transparency and data integrity. These occurrences underscore the critical importance of rigorous validation and verification processes in biodiversity studies, particularly when addressing unexpected findings in unique and sensitive environments like the Arctic. Ensuring the accuracy of species identification through meticulous peer review and cross-referencing with established databases is essential. Such occurrences, if confirmed, could indicate significant ecological shifts driven by climate change, emphasizing the necessity for continuous, comprehensive monitoring to accurately assess and understand the broader impacts on species distributions and ecosystem dynamics.
Approximately 50 % of all HA occurrences are primarily represented by only five species: Pseudo-nitzschia delicatissima (Cleve) Heiden, P. seriata (Cleve) H.Peragallo, Dinophysis acuminata Claparède & Lachmann, Prorocentrum cordatum (Ostenfeld) Dodge, and Mesodinium rubrum (Lohmann) Leegard (Fig. S5). Among these species, P. delicatissima, P. seriata, P. cordatum, and M. rubrum showcase an extensive geographic distribution, aligning with their broad prevalence across different regions, including the Arctic (Fig. S6; Bates et al., 2020, 2018; Lassus et al., 2016). In contrast, D. acuminata demonstrates a more confined geographic range (Fig. S6). While the presence of M. rubrum, which serves as prey for D. acuminata (Reguera et al., 2012), could potentially influence the distribution of this species, it cannot provide a comprehensive explanation for the constrained range of D. acuminata. This notion is underscored by the relatively broader geographic distribution observed for M. rubrum (Fig. S6). Temperature is also unlikely to be another restricting factor, as D. acuminata demonstrates tolerance to a temperature range from 4 to 10 °C, which corresponds to the temperatures observed in the region where this species occurred. The observed limited distribution of D. acuminata may therefore arise from a complex interplay of ecological and environmental factors that collectively shape its spatial pattern, a certainty that remains elusive in the scope of the present study. Additionally, this limited distribution could also be attributed to insufficient studies focused on this particular species.
Table 2Summary of the Spearman rank correlation (ρ) analysis between the maximum latitude of HA species for each month and year (max. LatHA) and the maximum latitude recorded for each month and year (max. Latrecorded) for each HA taxon.
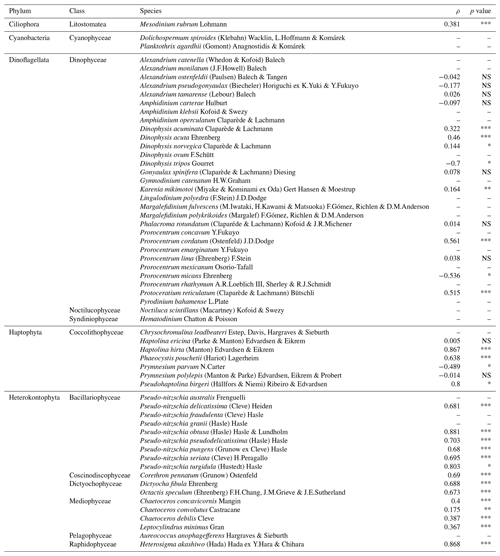
The significance is expressed as follows: NS, p value >0.05; * p value <0.05; ** p value <0.01; *** p value <0.001. The “–” symbol indicates not tested.
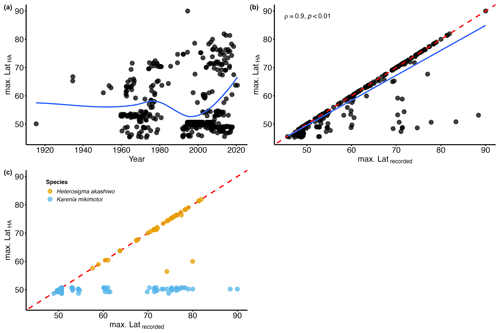
Figure 6(a) Temporal variation in the maximum latitude of HA species for each month and year (max. LatHA); the blue line represents the locally estimated scatterplot smoothing (LOESS). (b) Relationship between the maximum latitude of HA species for each month and year (max. LatHA) and the maximum latitude recorded for each month and year (max. Latrecorded); the blue line represents the linear model; the red dashed line represents the 1:1 slope. (c) Relationship between the maximum latitude of HA species for each month and year (max. LatHA) and the maximum latitude recorded for each month and year (max. Latrecorded) for Heterosigma akashiwo (yellow circle) and Karenia mikimotoi (blue circle); the red dashed line represents the 1:1 slope.
Climate change is expected to cause HA species to shift towards northern latitudes, increasing their prevalence in the North American Arctic region. In the Fram Strait, Nöthig et al. (2015) reported a dominance shift towards the harmful prymnesiophyte species Phaeocystis pouchetii, likely driven by a warm water anomaly in the Atlantic waters of the West Spitsbergen Current. Additionally, during a research cruise in the summer of 2022, an unprecedented bloom of Alexandrium catenella (Whedon & Kofoid) Balech, in terms of its scale, abundance, and toxicity, was tracked as it moved through the Bering Strait (Fachon et al., 2024). However, the extent of this northward progression of HA species in other Arctic regions, particularly the North American sector, remains relatively unexplored. To address this gap, we examined the temporal variation in the northernmost latitude where HA species are observed over the years. The analysis of the maximum latitude of HA species for each month and year (i.e. max. LatHA) reveals a gradual increase over time (Fig. 6a). To capture this trend more accurately, we applied locally estimated scatterplot smoothing (LOESS) regression. LOESS is particularly suitable for our dataset, which spans multiple decades and involves significant variability in sampling efforts. Unlike linear or polynomial regressions, LOESS does not assume a fixed functional form. Instead, it fits localized regressions to small subsets of the data, resulting in a smooth curve that better reflects the underlying patterns without imposing rigid assumptions about the data's structure. By using LOESS, we can visualize trends in the data while accounting for the inherent variability, allowing for a clearer picture of long-term patterns. The LOESS curve smooths out short-term fluctuations, revealing a marked increase towards the 1990s (Fig. 6a), emphasizing the accelerated northward progression of HA species in recent years. However, this trend is likely influenced by heightened oceanographic research and expeditions in higher latitudes, as evidenced by the strong correlation between max. LatHA and the maximum recorded latitude (max. Latrecorded; ρ=0.9; p value <0.01; Fig. 6b). Nonetheless, this association appears to exhibit variability depending on the species (Table 2). It is noteworthy that, among the species analysed, there are 24 species with insufficient available data to calculate the correlation (Table 2). For 12 species, there is a very weak Spearman rank correlation (; Table 2), indicating no meaningful linkage between max. LatHA and max. Latrecorded. One such example is the dinoflagellate species Karenia mikimotoi (Miyake & Kominami ex Oda) Hansen & Moestrup, which consistently maintains a near-constant max. LatHA despite an increasing max. Latrecorded (Fig. 6c). This pattern suggests that, while the sampling efforts expand northward, K. mikimotoi seems to be restricted to a specific latitude range. This limited latitudinal distribution is possibly attributed to its temperature tolerance range (4–30 °C) (Li et al., 2019). The colder temperatures in the North American Arctic align with the lower thermal limit of this species, likely acting as a thermal barrier to the dispersal of K. mikimotoi. Conversely, 12 species demonstrate a strong positive correlation (ρ>0.6; Table 2) that emphasizes a significant relationship between max. LatHA and max. Latrecorded. For instance, the max. LatHA of the raphidophyte species Heterosigma akashiwo (Hada) Hada ex Hara & Chihara appears to be closely linked to the max. Latrecorded (Fig. 6c; Table 2). This suggests the possibility of H. akashiwo being a permanent resident of the North American Arctic algal community. However, there remains uncertainty about whether the species observed in the database correspond to those found in temperate regions, as records of H. akashiwo in our database are identified with qualifiers such as cf. or aff. (Bérard-Therriault et al., 1999), indicating some uncertainty in their identification. In addition, Arctic conditions may not be conducive to its growth (Edvardsen and Imai, 2006; Mehdizadeh Allaf, 2023). In particular, toxin production is lowest at 30 °C, and blooms of H. akashiwo have been observed at temperatures ≥15 °C (Edvardsen and Imai, 2006; Mehdizadeh Allaf, 2023), suggesting that toxin production in the Arctic might be significantly reduced due to lower temperatures. The findings concerning the constrained latitudinal distribution of K. mikimotoi and the potential permanent residency of H. akashiwo in North American Arctic waters highlight the significance of investigating environmental factors and biological traits that shape the distribution and abundance of HA species in the Arctic Ocean and adjacent seas. In particular, gaining insights into thermal limits, growth requirements, and toxin production of these species can provide valuable information on their responses to the evolving Arctic climate and potential risks to human health and ecosystems. Further research is needed to investigate the population dynamics and ecological roles of these HA species within the Arctic context as well as their interactions with other marine organisms and the physical environment. In addition, utilizing molecular techniques to confirm the identity of these species would help clarify whether H. akashiwo is indeed a permanent resident of the North American Arctic algal community or if the records are due to misidentifications or persistent contamination.
While studies have detected phycotoxins in the North American Arctic (Baggesen et al., 2012; Elferink et al., 2017a; Gao et al., 2019; Hubbard et al., 2023; Li et al., 2016; Pućko et al., 2023), there are no reports of HA events at high latitudes (>60° N) in the Harmful Algal Event Database (HAEDAT; http://haedat.iode.org/index.php; last access: October 2023). HAEDAT's criteria for a HA event are strict, including toxin accumulation in seafood above safe levels; discolouration or scum in the water causing ecosystem or socioeconomic damage; negative effects on humans, animals, or other organisms; or precautionary closures of harvesting areas based on predefined thresholds of toxic phytoplankton cells in the water. This suggests that these events may not meet the HAEDAT criteria and raises questions about reconsidering and potentially revising the criteria used to monitor harmful algal blooms (HABs) in this unique region, particularly in the context of rapid environmental changes. The impacts of HABs in the Arctic may be more subtle, chronic, and ecosystem-specific; therefore, they might not trigger the HAEDAT thresholds designed for more temperate regions. For instance, the Arctic marine ecosystem is particularly sensitive to changes, and even low-level toxin presence can have significant impacts on local wildlife and indigenous communities that rely heavily on marine resources. To address these unique challenges, it may be important to develop Arctic-specific monitoring criteria that consider the distinct ecological and socioeconomic context of the region. Such criteria could include lower thresholds for toxin levels, more sensitive indicators of ecosystem health, and an emphasis on the cumulative effects of low-level exposures over time. Additionally, incorporating traditional ecological knowledge from indigenous communities could enhance monitoring efforts, as these communities have long-standing observations and insights into local environmental changes. Encouraging the adoption of these tailored criteria would support more comprehensive monitoring efforts, ensuring that all relevant harmful algal events are documented. This would not only improve our understanding of HABs in the Arctic but also inform better management and mitigation strategies to protect the Arctic marine ecosystem and the communities that depend on it.
The dataset described in this work is available from the Zenodo repository: https://doi.org/10.5281/zenodo.10498858 (Schiffrine et al., 2024).
The code used in this study is publicly accessible on Zenodo: https://doi.org/10.5281/zenodo.10498858 (Schiffrine et al., 2024). This repository contains the scripts and tools used for various aspects of our study, including data conversion, data quality control, analysis, and visualization.
Several databases exist that document the occurrence of microbial plankton taxa in temperate marine ecosystems. However, these resources often have limited representation of polar ecosystems or may lack such data entirely. Given the substantial environmental changes in the Arctic Ocean and their impact on microbial plankton communities, it is crucial to expand our understanding of Arctic phytoplankton biodiversity and biogeography. This study compiled various sources of digital biological records, both published and unpublished, to create a comprehensive dataset of North American Arctic marine microbial plankton occurrences. This dataset encompasses 385 348 individual georeferenced data points and 18 266 unique sampling events, covering 1422 species, including key phyla like Heterokontophyta, Dinoflagellata, Haptophyta, Ciliophora, and others. This effort addresses the historical limitations of Arctic microbial plankton data, which were often confined to specific regions or lacked comprehensive geographic and date-referenced records (Poulin et al., 2011).
Our study provides the largest database to date on the occurrence of microbial plankton species, including photoautotrophic prokaryotes and eukaryotes (i.e. phytoplankton), as well as hetero-, phago-, and mixotrophic protistan species in the North American part of the Arctic. This dataset can serve as a valuable resource for investigating the biogeography and phenology of microbial plankton taxa in the region, particularly when integrated with other published datasets. Through the application of geostatistical methods, our database contributes to a refined understanding of potential changes in Arctic microbial plankton communities in the future. Additionally, by supplementing our dataset with information concerning the toxicity or harmful nature of species, it facilitates assessments of the potential proliferation of toxic and/or harmful species within the Arctic Ocean.
Moreover, in light of the comprehensive analysis conducted in this study, it becomes evident that routine phycotoxin monitoring should encompass the North American Arctic. Historically, this region has been overlooked, driven by the assumption that high-latitude Arctic areas are not prone to significant toxic algal blooms or phycotoxin contamination. Nevertheless, our research, which sheds light on the diversity, distribution, and prevalence of HA species within this distinct area, emphatically underscores the urgency to reassess this perspective. The conspicuous presence of HA species underscores the imperative for a comprehensive and proactive monitoring strategy.
The supplement related to this article is available online at: https://doi.org/10.5194/essd-16-5681-2024-supplement.
NS led the study, the data processing and archiving, and the writing. FD, MP, SL, AR, and MG provided the quality-controlled data from different Arctic regions. KD initiated the collection of data in Iqaluit and surrounding areas of Frobisher Bay as part of the Baseline Program in 2019. All authors reviewed and commented on the manuscript.
The contact author has declared that none of the authors has any competing interests.
Publisher's note: Copernicus Publications remains neutral with regard to jurisdictional claims made in the text, published maps, institutional affiliations, or any other geographical representation in this paper. While Copernicus Publications makes every effort to include appropriate place names, the final responsibility lies with the authors.
The authors are grateful to local community members, Fisheries and Oceans Canada, and university collaborators, who participated in sampling and taxonomic analysis. We thank the AlgaeBase team for their invaluable assistance with respect to providing us with an API key. This is a contribution to the research programs of the Institut des sciences de la mer of UQAR and Québec-Océan.
This research has been supported by the Ocean Protection Plan and Ocean and Freshwater Science Contribution Program of Fisheries and Oceans Canada (grant no. 19-005-CA-UQAR), the Natural Sciences and Engineering Research Council of Canada (grant nos. RGPIN/6437-2017, RGPNS/305492-85 2017, and RGPNS/305501-2019), the Network of Centres of Excellence of Canada ArcticNet, and the Fonds de recherche du Québec – Nature et technologies through the strategic cluster Québec-Océan (grant nos. 2016-RS-186795 and 2023-RS6-309012) to Michel Gosselin and André Rochon.
This paper was edited by Frédéric Gazeau and reviewed by two anonymous referees.
Ahyong, S., Boyko, C. B., Bernot, J., Brandão, S. N., Daly, M., De Grave, S., de Voogd, N. J., Gofas, S., Hernandez, F., Hughes, L., Neubauer, T. A., Paulay, G., van der Meij, S., Boydens, B., Decock, W., Dekeyzer, S., Goharimanesh, M., Vandepitte, L., Vanhoorne, B., Adlard, R., Agatha, S., Ahn, K. J., Alonso, M. V., Alvarez, B., Amler, M. R. W., Amorim, V., Anderberg, A., Andrés-Sánchez, S., Ang, Y., Antić, D., Antonietto, L. S., Arango, C., Artois, T., Atkinson, S., Auffenberg, K., Bailly, N., Baldwin, B. G., Bank, R., Barber, A., Barrett, R. L., Bartsch, I., Bellan-Santini, D., Bergh, N., Bernard, C., Berrios Ortega, F. J., Berta, A., Bezerra, T. N., Bieler, R., Blanco, S., Blasco-Costa, I., Blazewicz, M., Błędzki, L. A., Bock, P., Bonifacino, M., Böttger-Schnack, R., Bouchet, P., Boury-Esnault, N., Bouzan, R., Boxshall, G., Bray, R., Brito Seixas, A. L., Bruce, N. L., Bruneau, A., Budaeva, N., Bueno-Villegas, J., Calvo Casas, J., Cárdenas, P., Carstens, E., Cartwright, P., Cedhagen, T., Chan, B. K., Chan, T. Y., Chernyshev, A., Choong, H., Christenhusz, M., Churchill, M., Collins, A. G., Collins, G. E., Collins, K., Consorti, L., Copilaș-Ciocianu, D., Corbari, L., Cordeiro, R., Costa, V. M. D. M., Costa Corgosinho, P. H., Coste, M., Costello, M. J., Crandall, K. A., Cremonte, F., Cribb, T., Cuscó-Borràs, J., Cutmore, S., Dahdouh-Guebas, F., Daneliya, M., Dauvin, J. C., Davie, P., De Broyer, C., de Lima Ferreira, P., de Mazancourt, V., de Moura Oliveira, L., Decker, P., Defaye, D., Dekker, H., Di Capua, I., Dippenaar, S., Dohrmann, M., Dolan, J., Domning, D., Downey, R., Dreyer, N., Eisendle, U., Eitel, M., Eleaume, M., Enghoff, H., Epler, J., Esquete Garrote, P., Evenhuis, N. L., Ewers-Saucedo, C., Faber, M., Figueroa, D., Fišer, C., Fordyce, E., Foster, W., Fransen, C., Freire, S., Fujimoto, S., Furuya, H., Galbany-Casals, M., Gale, A., Galea, H., Gao, T., García-Moro, P., Garic, R., Garnett, S., Gaviria-Melo, S., Gerken, S., Gibson, D., Gibson, R., Gil, J., Gittenberger, A., Glasby, C., Glenner, H., Glover, A., Gómez, I., Gómez-Noguera, S. E., Gondim, A. I., Gonzalez, B. C., González-Solís, D., Goodwin, C., Gostel, M., Grabowski, M., Gravili, C., Grossi, M., Guerra-García, J. M., Guerrero, J. M., Guidetti, R., Guiry, M. D., Gutierrez, D., Hadfield, K. A., Hajdu, E., Halanych, K., Hallermann, J., Hayward, B. W., Hegna, T. A., Heiden, G., Hendrycks, E., Hennen, D., Herbert, D., Herrera Bachiller, A., Hodda, M., Høeg, J., Hoeksema, B., Holovachov, O., Hooge, M. D., Hooper, J. N., Horton, T., Houart, R., Huys, R., Hyžný, M., Iniesta, L. F. M., Iseto, T., Iwataki, M., Janssen, R., Jaume, D., Jazdzewski, K., Jersabek, C. D., Jiménez-Mejías, P., Jóźwiak, P., Kabat, A., Kajihara, H., Kakui, K., Kantor, Y., Karanovic, I., Karapunar, B., Karthick, B., Kathirithamby, J., Katinas, L., Kilian, N., Kim, Y. H., King, R., Kirk, P. M., Klautau, M., Kociolek, J. P., Köhler, F., Konowalik, K., Kotov, A., Kovács, Z., Kremenetskaia, A., Kristensen, R. M., Kroh, A., Kulikovskiy, M., Kullander, S., Kupriyanova, E., Lamaro, A., Lambert, G., Larridon, I., Lazarus, D., Le Coze, F., Le Roux, M., LeCroy, S., Leduc, D., Lefkowitz, E. J., Lemaitre, R., Lichter-Marck, I. H., Lim, S. C., Lindsay, D., Liu, Y., Loeuille, B., Lois, R., Lörz, A. N., Ludwig, T., Lundholm, N., Macpherson, E., Maguilla, E., Mah, C., Mamos, T., Manconi, R., Mapstone, G., Marek, P. E., Markello, K., Márquez-Corro, J. I., Marshall, B., Marshall, D. J., Martin, P., Martín-Bravo, S., Martinez Arbizu, P., Maslakova, S., McFadden, C., McInnes, S. J., McKenzie, R., Means, J., Mees, J., Mejía-Madrid, H. H., Meland, K., Merrin, K. L., Míguez, M., Miller, J., Mills, C., Moestrup, Ø., Mokievsky, V., Molodtsova, T., Monniot, F., Mooi, R., Morales-Alonso, A., Morandini, A. C., Moreira da Rocha, R., Morrow, C., Mortelmans, J., Müller, A., Muñoz Gallego, A. R., Muñoz Schüler, P., Musco, L., Nascimento, J. B., Nesom, G., Neto Silva, M. D. S., Neubert, E., Neuhaus, B., Ng, P., Nguyen, A. D., Nielsen, C., Nielsen, S., Nishikawa, T., Norenburg, J., O'Hara, T., Opresko, D., Osawa, M., Osigus, H. J., Ota, Y., Páll-Gergely, B., Panero, J. L., Patterson, D., Pedram, M., Pelser, P., Peña Santiago, R., Perbiche-Neves, G., Pereira, J. D. S., Pereira, P. H. M., Pereira, S. G. G., Perez-Losada, M., Petrescu, I., Pfingstl, T., Piasecki, W., Pica, D., Picton, B., Pignatti, J., Pilger, J. F., Pinheiro, U., Pisera, A. B., Poatskievick Pierezan, B., Polhemus, D., Poore, G. C., Potapova, M., Praxedes, R. A., Půža, V., Read, G., Reich, M., Reimer, J. D., Reip, H., Resende Bueno, V., Reuscher, M., Reynolds, J. W., Richling, I., Rimet, F., Ríos, P., Rius, M., Rodríguez, E., Rogers, D. C., Roque, N., Rosenberg, G., Rützler, K., Sa, I. R. D. S., Sá, H. A. B., Saavedra, M., Sabater, L. M., Sabbe, K., Sabroux, R., Saiz-Salinas, J., Sala, S., Samimi-Namin, K., Sánchez-Villegas, M., Sánchez-Villegas, R., Santagata, S., Santos, S., Santos, S. G., Santos Filho, M. A. B. D., Sanz Arnal, M., Sar, E., Saucède, T., Schärer, L., Schierwater, B., Schilling, E., Schmidt-Lebuhn, A., Schneider, S., Schönberg, C., Schrével, J., Schuchert, P., Schweitzer, C., Semple, J. C., Senna, A. R., Sennikov, A., Serejo, C., Shaik, S., Shamsi, S., Sharma, J., Shear, W. A., Shenkar, N., Short, M., Sicinski, J., Sierwald, P., Silva, D. K. F. D., Silva, E. S. S., Silva, M. L. C. N., Simmons, E., Sinniger, F., Sinou, C., Sivell, D., Smit, H., Smit, N., Smol, N., Sørensen, M. V., Souza-Filho, J. F., Spelda, J., Sterrer, W., Steyn, H. M., Stoev, P., Stöhr, S., Suárez-Morales, E., Susanna, A., Suttle, C., Swalla, B. J., Taiti, S., Tanaka, M., Tandberg, A. H., Tang, D., Tasker, M., Taylor, J., Taylor, J., Taylor, K., Tchesunov, A., Temereva, E., ten Hove, H., ter Poorten, J. J., Thirouin, K., Thomas, J. D., Thuesen, E. V., Thurston, M., Thuy, B., Timi, J. T., Todaro, A., Todd, J., Turon, X., Tyler, S., Uetz, P., Urbatsch, L., Uribe-Palomino, J., Urtubey, E., Utevsky, S., Vacelet, J., Vader, W., Väinölä, R., Valdés-Florido, A., Valls Domedel, G., Van de Vijver, B., van Haaren, T., van Soest, R. W., Vanreusel, A., Vázquez-García, B., Velandia, J., Venekey, V., Verhoeff, T., Villaverde, T., Vinarski, M., Vonk, R., Vos, C., Vouilloud, A. A., Walker-Smith, G., Walter, T. C., Watling, L., Wayland, M., Wesener, T., Wetzel, C. E., Whipps, C., White, K., Wieneke, U., Williams, D. M., Williams, G., Williams, N., Wilson, K. L., Wilson, R., Witkowski, J., Wyatt, N., Xavier, J., Xu, K., Zanol, J., Zeidler, W., Zhao, Z., and Zullini, A.: World Register of Marine Species (WoRMS), https://doi.org/10.14284/170, 2023.
AlgaeBase: World-wide electronic publication, https://www.algaebase.org/about/, last access: 22 June 2023.
AMAP: AMAP Assessment 2018: Arctic Ocean Acidification, Arctic Monitoring and Assessment Programme (AMAP), Tromsø, Norway, vi+187 pp., 2018.
Anderson, D. M., Fachon, E., Pickart, R. S., Lin, P., Fischer, A. D., Richlen, M. L., Uva, V., Brosnahan, M. L., McRaven, L., Bahr, F., Lefebvre, K., Grebmeier, J. M., Danielson, S. L., Lyu, Y., and Fukai, Y.: Evidence for massive and recurrent toxic blooms of Alexandrium catenella in the Alaskan Arctic, P. Natl. Acad. Sci. USA, 118, e2107387118, https://doi.org/10.1073/pnas.2107387118, 2021.
Archambault, P., Snelgrove, P. V. R., Fisher, J. A. D., Gagnon, J.-M., Garbary, D. J., Harvey, M., Kenchington, E. L., Lesage, V., Levesque, M., Lovejoy, C., Mackas, D. L., McKindsey, C. W., Nelson, J. R., Pepin, P., Piché, L., and Poulin, M.: From Sea to Sea: Canada's Three Oceans of Biodiversity, PLoS ONE, 5, e12182, https://doi.org/10.1371/journal.pone.0012182, 2010.
Ardyna, M. and Arrigo, K. R.: Phytoplankton dynamics in a changing Arctic Ocean, Nat. Clim. Change, 10, 892–903, https://doi.org/10.1038/s41558-020-0905-y, 2020.
Ardyna, M., Babin, M., Gosselin, M., Devred, E., Rainville, L., and Tremblay, J.-É.: Recent Arctic Ocean sea ice loss triggers novel fall phytoplankton blooms, Geophys. Res. Lett., 41, 6207–6212, https://doi.org/10.1002/2014GL061047, 2014.
Ardyna, M., Babin, M., Devred, E., Forest, A., Gosselin, M., Raimbault, P., and Tremblay, J.-É.: Shelf-basin gradients shape ecological phytoplankton niches and community composition in the coastal Arctic Ocean (Beaufort Sea), Limnol. Oceanogr., 62, 2113–2132, https://doi.org/10.1002/lno.10554, 2017.
Baggesen, C., Moestrup, Ø., Daugbjerg, N., Krock, B., Cembella, A. D., and Madsen, S.: Molecular phylogeny and toxin profiles of Alexandrium tamarense (Lebour) Balech (Dinophyceae) from the west coast of Greenland, Harmful Algae, 19, 108–116, https://doi.org/10.1016/j.hal.2012.06.005, 2012.
Balzano, S., Marie, D., Gourvil, P., and Vaulot, D.: Composition of the summer photosynthetic pico and nanoplankton communities in the Beaufort Sea assessed by T-RFLP and sequences of the 18S rRNA gene from flow cytometry sorted samples, The ISME Journal, 6, 1480–1498, https://doi.org/10.1038/ismej.2011.213, 2012.
Barnes, R. and Sahr, K.: dggridR: Discrete Global Grids, https://github.com/r-barnes/dggridR (last access: 27 August 2024), 2020.
Baselga, A.: Partitioning the turnover and nestedness components of beta diversity, Global Ecol. Biogeogr., 19, 134–143, https://doi.org/10.1111/j.1466-8238.2009.00490.x, 2010.
Baselga, A. and Orme, C. D. L.: betapart: an R package for the study of beta diversity, Methods Ecol. Evol., 3, 808–812, https://doi.org/10.1111/j.2041-210X.2012.00224.x, 2012.
Bates, S., Beach, D., Comeau, L., Haigh, N., Lewis, N., Locke, A., Martin, J., McCarron, P., Mckenzie, C., Michel, C., Miles, C., Poulin, M., Quilliam, M., Rourke, W., Scarratt, M., Starr, M., and Wells, T.: Marine harmful algal blooms and phycotoxins of concern to Canada, Canadian Technical Report of Fisheries and Aquatic Sciences, 3384, x+322, 2020.
Bates, S. S., Hubbard, K. A., Lundholm, N., Montresor, M., and Leaw, C. P.: Pseudo-nitzschia, Nitzschia, and domoic acid: New research since 2011, Harmful Algae, 79, 3–43, https://doi.org/10.1016/j.hal.2018.06.001, 2018.
Bates, S. S., Lundholm, N., Hubbard, K. A., Montresor, M., and Leaw, C. P.: Toxic and Harmful Marine Diatoms, in: Diatoms: Fundamentals and Applications, John Wiley & Sons, Ltd, 389–434, https://doi.org/10.1002/9781119370741.ch17, 2019.
Bendif, E. M., Nevado, B., Wong, E. L. Y., Hagino, K., Probert, I., Young, J. R., Rickaby, R. E. M., and Filatov, D. A.: Repeated species radiations in the recent evolution of the key marine phytoplankton lineage Gephyrocapsa, Nat. Commun., 10, 4234, https://doi.org/10.1038/s41467-019-12169-7, 2019.
Bendif, E. M., Probert, I., Archontikis, O. A., Young, J. R., Beaufort, L., Rickaby, R. E., and Filatov, D.: Rapid diversification underlying the global dominance of a cosmopolitan phytoplankton, The ISME Journal, 17, 630–640, https://doi.org/10.1038/s41396-023-01365-5, 2023.
Benedetti, F., Vogt, M., Elizondo, U. H., Righetti, D., Zimmermann, N. E., and Gruber, N.: Major restructuring of marine plankton assemblages under global warming, Nat. Commun., 12, 5226, https://doi.org/10.1038/s41467-021-25385-x, 2021.
Bérard-Therriault, L., Poulin, M., and Bossé, L.: Guide d'identification du phytoplancton marin de l'estuaire et du golfe du Saint-Laurent incluant également certains protozoaires, Publication spéciale canadienne des sciences halieutiques et aquatiques, Ottawa, Ont., 387 pp., 1999.
Blais, M., Ardyna, M., Gosselin, M., Dumont, D., Bélanger, S., Tremblay, J.-É., Gratton, Y., Marchese, C., and Poulin, M.: Contrasting interannual changes in phytoplankton productivity and community structure in the coastal Canadian Arctic Ocean, Limnol. Oceanogr., 62, 2480–2497, https://doi.org/10.1002/lno.10581, 2017.
Bruhn, C. S., Wohlrab, S., Krock, B., Lundholm, N., and John, U.: Seasonal plankton succession is in accordance with phycotoxin occurrence in Disko Bay, West Greenland, Harmful Algae, 103, 101978, https://doi.org/10.1016/j.hal.2021.101978, 2021.
Buitenhuis, E. T., Li, W. K. W., Lomas, M. W., Karl, D. M., Landry, M. R., and Jacquet, S.: Picoheterotroph (Bacteria and Archaea) biomass distribution in the global ocean, Earth Syst. Sci. Data, 4, 101–106, https://doi.org/10.5194/essd-4-101-2012, 2012.
Carmack, E. C., Yamamoto-Kawai, M., Haine, T. W. N., Bacon, S., Bluhm, B. A., Lique, C., Melling, H., Polyakov, I. V., Straneo, F., Timmermans, M. L., and Williams, W. J.: Freshwater and its role in the Arctic Marine System: Sources, disposition, storage, export, and physical and biogeochemical consequences in the Arctic and global oceans, J. Geophys. Res.-Biogeo., 121, 675–717, https://doi.org/10.1002/2015JG003140, 2016.
Chamberlain, S. and Vanhoorne, B.: worrms: World Register of Marine Species (WoRMS) Client [R package], R package version 0.4.3, https://CRAN.R-project.org/package=worrms (last access: 27 August 2024), 2023.
Chamberlain, S., Schepers, L., and Fernandez, S.: mregions: Marine Regions Data from “Marineregions.org” [R package], R package version 0.1.9, https://github.com/ropensci/mregions (last access: 27 August 2024), 2024.
Chan, F. T., Stanislawczyk, K., Sneekes, A. C., Dvoretsky, A., Gollasch, S., Minchin, D., David, M., Jelmert, A., Albretsen, J., and Bailey, S. A.: Climate change opens new frontiers for marine species in the Arctic: Current trends and future invasion risks, Global Change Biol., 25, 25–38, https://doi.org/10.1111/gcb.14469, 2019.
Chao, A. and Shen, T.-J.: Nonparametric estimation of Shannon's index of diversity when there are unseen species in sample, Environ. Ecol. Stat., 10, 429–443, https://doi.org/10.1023/A:1026096204727, 2003.
Chaudhary, C., Saeedi, H., and Costello, M. J.: Bimodality of Latitudinal Gradients in Marine Species Richness, Trends Ecol. Evol., 31, 670–676, https://doi.org/10.1016/j.tree.2016.06.001, 2016.
Chaudhary, C., Saeedi, H., and Costello, M. J.: Marine Species Richness Is Bimodal with Latitude: A Reply to Fernandez and Marques, Trends Ecol. Evol., 32, 234–237, https://doi.org/10.1016/j.tree.2017.02.007, 2017.
Codispoti, L. A., Kelly, V., Thessen, A., Matrai, P., Suttles, S., Hill, V., Steele, M., and Light, B.: Synthesis of primary production in the Arctic Ocean: III. Nitrate and phosphate based estimates of net community production, Prog. Oceanogr., 110, 126–150, https://doi.org/10.1016/j.pocean.2012.11.006, 2013.
Comeau, A. M., Li, W. K. W., Tremblay, J.-É., Carmack, E. C., and Lovejoy, C.: Arctic Ocean Microbial Community Structure before and after the 2007 Record Sea Ice Minimum, PLOS ONE, 6, e27492, https://doi.org/10.1371/journal.pone.0027492, 2011.
Coupel, P., Matsuoka, A., Ruiz-Pino, D., Gosselin, M., Marie, D., Tremblay, J.-É., and Babin, M.: Pigment signatures of phytoplankton communities in the Beaufort Sea, Biogeosciences, 12, 991–1006, https://doi.org/10.5194/bg-12-991-2015, 2015.
Dhifallah, F., Rochon, A., Simard, N., McKindsey, C. W., Gosselin, M., and Howland, K. L.: Dinoflagellate communities in high-risk Canadian Arctic ports, Estuarine, Coast. Shelf Sci., 266, 107731, https://doi.org/10.1016/j.ecss.2021.107731, 2021.
Edvardsen, B. and Imai, I.: The ecology of harmful flagellates within Prymnesiophyceae and Raphidophyceae, in: Ecology of Harmful Algae, edited by: Granéli, E. and Turner, J. T., Springer, Berlin, Heidelberg, 67–79, https://doi.org/10.1007/978-3-540-32210-8_6, 2006.
Elferink, S., Neuhaus, S., Wohlrab, S., Toebe, K., Voß, D., Gottschling, M., Lundholm, N., Krock, B., Koch, B. P., Zielinski, O., Cembella, A., and John, U.: Molecular diversity patterns among various phytoplankton size-fractions in West Greenland in late summer, Deep-Sea Res. Pt. I, 121, 54–69, https://doi.org/10.1016/j.dsr.2016.11.002, 2017a.
Elferink, S., Neuhaus, S., Wohlrab, S., Toebe, K., Voß, D., Gottschling, M., Lundholm, N., Krock, B., Koch, B. P., Zielinski, O., Cembella, A., and John, U.: Operational taxonomic units and taxonomy of a phytoplankton community during Maria S. Merian cruise MSM21/3 – Greenland (ARCHEMHAB), PANGAEA [data set], https://doi.org/10.1594/PANGAEA.857405, 2017b.
Fachon, E., Pickart, R. S., Sheffield, G., Pate, E., Pathare, M., Brosnahan, M. L., Muhlbach, E., Horn, K., Spada, N. N., Rajagopalan, A., Lin, P., McRaven, L. T., Lago, L. S., Huang, J., Bahr, F., Stockwell, D. A., Hubbard, K. A., Farrugia, T. J., Lefebvre, K. A., and Anderson, D. M.: Tracking a large-scale and highly toxic Arctic algal bloom: Rapid detection and risk communication, Limnol. Oceanogr. Lett., https://doi.org/10.1002/lol2.10421, 2024.
Flombaum, P., Gallegos, J. L., Gordillo, R. A., Rincón, J., Zabala, L. L., Jiao, N., Karl, D. M., Li, W. K. W., Lomas, M. W., Veneziano, D., Vera, C. S., Vrugt, J. A., and Martiny, A. C.: Present and future global distributions of the marine Cyanobacteria Prochlorococcus and Synechococcus, P. Natl. Acad. Sci. USA, 110, 9824–9829, https://doi.org/10.1073/pnas.1307701110, 2013.
Freyria, N. J., Joli, N., and Lovejoy, C.: A decadal perspective on north water microbial eukaryotes as Arctic Ocean sentinels, Sci. Rep., 11, 8413, https://doi.org/10.1038/s41598-021-87906-4, 2021.
Gao, C., Lin, S., Chen, M., Hong, J., and Liu, C.: Prevalence of phycotoxin contamination in shellfish from the Northern Bering Sea and the Chukchi Sea, Toxicon, 167, 76–81, https://doi.org/10.1016/j.toxicon.2019.06.001, 2019.
Guiry, M. D., Moestrup, Ø., and Andersen, R. A.: Validation of the phylum name Heterokontophyta, Notulae Algarum, 297, 1–5, 2023.
Hallegraeff, G. M., Anderson, D. M., Belin, C., Bottein, M.-Y. D., Bresnan, E., Chinain, M., Enevoldsen, H., Iwataki, M., Karlson, B., McKenzie, C. H., Sunesen, I., Pitcher, G. C., Provoost, P., Richardson, A., Schweibold, L., Tester, P. A., Trainer, V. L., Yñiguez, A. T., and Zingone, A.: Perceived global increase in algal blooms is attributable to intensified monitoring and emerging bloom impacts, Commun. Earth Environ., 2, 1–10, https://doi.org/10.1038/s43247-021-00178-8, 2021.
Hanna, E., Nolan, J. E., Overland, J. E., and Hall, R. J.: Climate Change in the Arctic, in: Arctic Ecology, John Wiley & Sons, Ltd, 57–79, https://doi.org/10.1002/9781118846582.ch3, 2021.
Hörstmann, C., Hattermann, T., Thomé, P. C., Buttigieg, P. L., Morel, I., Waite, A. M., and John, U.: Biogeographic gradients of picoplankton diversity indicate increasing dominance of prokaryotes in warmer Arctic fjords, Commun. Biol., 7, 1–11, https://doi.org/10.1038/s42003-024-05946-8, 2024.
Hubbard, K. A., Villac, M. C., Chadwick, C., DeSmidt, A. A., Flewelling, L., Granholm, A., Joseph, M., Wood, T., Fachon, E., Brosnahan, M. L., Richlen, M., Pathare, M., Stockwell, D., Lin, P., Bouchard, J. N., Pickart, R., and Anderson, D. M.: Spatiotemporal transitions in Pseudo-nitzschia species assemblages and domoic acid along the Alaska coast, PLoS ONE, 18, e0282794, https://doi.org/10.1371/journal.pone.0282794, 2023.
Ibarbalz, F. M., Henry, N., Brandão, M. C., Martini, S., Busseni, G., Byrne, H., Coelho, L. P., Endo, H., Gasol, J. M., Gregory, A. C., Mahé, F., Rigonato, J., Royo-Llonch, M., Salazar, G., Sanz-Sáez, I., Scalco, E., Soviadan, D., Zayed, A. A., Zingone, A., Labadie, K., Ferland, J., Marec, C., Kandels, S., Picheral, M., Dimier, C., Poulain, J., Pisarev, S., Carmichael, M., Pesant, S., Tara Oceans Coordinators, Babin, M., Boss, E., Iudicone, D., Jaillon, O., Acinas, S. G., Ogata, H., Pelletier, E., Stemmann, L., Sullivan, M. B., Sunagawa, S., Bopp, L., de Vargas, C., Karp-Boss, L., Wincker, P., Lombard, F., Bowler, C., and Zinger, L.: Global Trends in Marine Plankton Diversity across Kingdoms of Life, Cell, 179, 1084–1097.e21, https://doi.org/10.1016/j.cell.2019.10.008, 2019.
Ibarbalz, F. M., Henry, N., Mahé, F., Ardyna, M., Zingone, A., Scalco, E., Lovejoy, C., Lombard, F., Jaillon, O., Iudicone, D., Malviya, S., Tara Oceans Coordinators, Sullivan, M. B., Chaffron, S., Karsenti, E., Babin, M., Boss, E., Wincker, P., Zinger, L., de Vargas, C., Bowler, C., and Karp-Boss, L.: Pan-Arctic plankton community structure and its global connectivity, Elementa, 11, 00060, https://doi.org/10.1525/elementa.2022.00060, 2023.
Joli, N., Gosselin, M., Ardyna, M., Babin, M., Onda, D. F., Tremblay, J.-É., and Lovejoy, C.: Need for focus on microbial species following ice melt and changing freshwater regimes in a Janus Arctic Gateway, Sci. Rep., 8, 1–11, https://doi.org/10.1038/s41598-018-27705-6, 2018.
Kacimi, S. and Kwok, R.: Arctic snow depth, ice thickness, and volume from ICESat-2 and CryoSat: 2018–2021, Geophys. Res. Lett., 49, e2021GL097448, https://doi.org/10.1029/2021GL097448, 2022.
Kahru, M., Brotas, V., Manzano-Sarabia, M., and Mitchell, B. G.: Are phytoplankton blooms occurring earlier in the Arctic?, Global Change Biol., 17, 1733–1739, https://doi.org/10.1111/j.1365-2486.2010.02312.x, 2011.
Kalenitchenko, D., Joli, N., Potvin, M., Tremblay, J.-É., and Lovejoy, C.: Biodiversity and Species Change in the Arctic Ocean: A View Through the Lens of Nares Strait, Front. Marine Sci., 6, 479, https://doi.org/10.3389/fmars.2019.00479, 2019.
Lassus, P., Chomérat, N., Hess, P., and Nézan, E.: Toxic and harmful microalgae of the World Ocean/Micro-algues toxiques et nuisibles de l'océan mondial, International Society for the Study of Harmful Algae/Intergovernmental Oceanographic Commission of UNESCO, Denmark, 523 pp., 2016.
Li, A., Chen, H., Qiu, J., Lin, H., and Gu, H.: Determination of multiple toxins in whelk and clam samples collected from the Chukchi and Bering seas, Toxicon, 109, 84–93, https://doi.org/10.1016/j.toxicon.2015.11.016, 2016.
Li, W. K. W., McLaughlin, F. A., Lovejoy, C., and Carmack, E. C.: Smallest Algae Thrive As the Arctic Ocean Freshens, Science, 326, 539–539, https://doi.org/10.1126/science.1179798, 2009.
Li, X., Yan, T., Yu, R., and Zhou, M.: A review of Karenia mikimotoi: Bloom events, physiology, toxicity and toxic mechanism, Harmful Algae, 90, 101702, https://doi.org/10.1016/j.hal.2019.101702, 2019.
Lovejoy, C., Galand, P. E., and Kirchman, D. L.: Picoplankton diversity in the Arctic Ocean and surrounding seas, Mar. Biodiv., 41, 5–12, https://doi.org/10.1007/s12526-010-0062-z, 2011.
Lovejoy, C., von Quillfeldt, C., Hopcroft, R. R., Poulin, M., Thaler, M., Arendt, K., Debes, H., Gíslason, Á., and Kosobokova, K.: State of the Arctic Marine Biodiversity Report: chapter 3.2: Plankton, in: State of the Arctic Marine Biodiversity Report, 63–83, 2017.
Lundholm, N., Churro, C., Escalera, L., Fraga, S., Hoppenrath, M., Iwataki, M., Larsen, J., Mertens, K., Moestrup, Ø., and Zingone, A.: IOC-UNESCO Taxonomic Reference List of Harmful Micro Algae, https://doi.org/10.14284/362, 2009.
Martin, J., Tremblay, J. É., and Price, N. M.: Nutritive and photosynthetic ecology of subsurface chlorophyll maxima in Canadian Arctic waters, Biogeosciences, 9, 5353–5371, https://doi.org/10.5194/bg-9-5353-2012, 2012.
McKenzie, C. H., Bates, S. S., Martin, J. L., Haigh, N., Howland, K. L., Lewis, N. I., Locke, A., Peña, A., Poulin, M., Rochon, A., Rourke, W. A., Scarratt, M. G., Starr, M., and Wells, T.: Three decades of Canadian marine harmful algal events: Phytoplankton and phycotoxins of concern to human and ecosystem health, Harmful Algae, 102, 101852, https://doi.org/10.1016/j.hal.2020.101852, 2020.
Mehdizadeh Allaf, M.: Heterosigma akashiwo, a Fish-Killing Flagellate, Microb. Res., 14, 132–147, https://doi.org/10.3390/microbiolres14010012, 2023.
Meredith, M., Sommerkorn, M., Cassotta, S., Derksen, C., Ekaykin, A., Hollowed, A., Kofinas, G., Mackintosh, A., Melbourne-Thomas, J., Muelbert, M. M. C., Ottersen, G., Pritchard, H., and Schuur, E. A. G.: Polar Regions, in: IPCC Special Report on the Ocean and Cryosphere in a Changing Climate, edited by: Pörtner, H.-O., Roberts, D. C., Masson-Delmotte, V., Zhai, P., Tignor, M., Poloczanska, E., Mintenbeck, K., Alegría, A., Nicolai, M., Okem, A., Petzold, J., Rama, B., Weyer, N. M., Cambridge University Press, Cambridge, UK and New York, NY, USA, 203–320, https://doi.org/10.1017/9781009157964.005, 2019.
Mitchell, A. L., Almeida, A., Beracochea, M., Boland, M., Burgin, J., Cochrane, G., Crusoe, M. R., Kale, V., Potter, S. C., Richardson, L. J., Sakharova, E., Scheremetjew, M., Korobeynikov, A., Shlemov, A., Kunyavskaya, O., Lapidus, A., and Finn, R. D.: MGnify: the microbiome analysis resource in 2020, Nucleic Acids Res., 48, D570–D578, https://doi.org/10.1093/nar/gkz1035, 2020.
Morquecho, L.: Pyrodinium bahamense One the Most Significant Harmful Dinoflagellate in Mexico, Front. Marine Sci., 6, 1, https://doi.org/10.3389/fmars.2019.00001, 2019.
Neukermans, G., Oziel, L., and Babin, M.: Increased intrusion of warming Atlantic water leads to rapid expansion of temperate phytoplankton in the Arctic, Global Change Biol., 24, 2545–2553, 2018.
Nohe, A., Goffin, A., Tyberghein, L., Lagring, R., De Cauwer, K., Vyverman, W., and Sabbe, K.: Marked changes in diatom and dinoflagellate biomass, composition and seasonality in the Belgian Part of the North Sea between the 1970s and 2000s, Sci. Total Environ., 716, 136316, https://doi.org/10.1016/j.scitotenv.2019.136316, 2020.
Nöthig, E.-M., Bracher, A., Engel, A., Metfies, K., Niehoff, B., Peeken, I., Bauerfeind, E., Cherkasheva, A., Gäbler-Schwarz, S., Hardge, K., Kilias, E., Kraft, A., Mebrahtom Kidane, Y., Lalande, C., Piontek, J., Thomisch, K., and Wurst, M.: Summertime plankton ecology in Fram Strait – a compilation of long- and short-term observations, Polar Res., 34, 23349, https://doi.org/10.3402/polar.v34.23349, 2015.
Okolodkov, Y. B. and Dodge, J. D.: Biodiversity and biogeography of planktonic dinoflagellates in the Arctic Ocean, J. Exp. Marine Biol. Ecol., 202, 19–27, https://doi.org/10.1016/0022-0981(96)00028-7, 1996.
Oksanen, J., Simpson, G., Blanchet, F., Kindt, R., Legendre, P., Minchin, P., O'Hara, R., Solymos, P., Stevens, M., Szoecs, E., Wagner, H., Barbour, M., Bedward, M., Bolker, B., Borcard, D., Carvalho, G., Chirico, M., De Caceres, M., Durand, S., Evangelista, H., FitzJohn, R., Friendly, M., Furneaux, B., Hannigan, G., Hill, M., Lahti, L., McGlinn, D., Ouellette, M., Ribeiro Cunha, E., Smith, T., Stier, A., Ter Braak, C., Weedon, J.: _vegan:Community Ecology Package [R package], R package version 2.6-8, https://CRAN.R-project.org/package=vegan (last access: 27 August 2024), 2024.
Olsen, L. M., Duarte, P., Peralta-Ferriz, C., Kauko, H. M., Johansson, M., Peeken, I., Różańska-Pluta, M., Tatarek, A., Wiktor, J., Fernández-Méndez, M., Wagner, P. M., Pavlov, A. K., Hop, H., and Assmy, P.: A red tide in the pack ice of the Arctic Ocean, Sci. Rep., 9, 9536, https://doi.org/10.1038/s41598-019-45935-0, 2019.
Oziel, L., Baudena, A., Ardyna, M., Massicotte, P., Randelhoff, A., Sallée, J.-B., Ingvaldsen, R. B., Devred, E., and Babin, M.: Faster Atlantic currents drive poleward expansion of temperate phytoplankton in the Arctic Ocean, Nat. Commun., 11, 1705, https://doi.org/10.1038/s41467-020-15485-5, 2020.
PAME: Large Marine Ecosystems (LMEs) of the Arctic area: revision of the Arctic LME map 15th of May 2013, Akureyri, Iceland: Conservation of Arctic Flora and Fauna (CAFF) and Protection of theArctic Marine Environment (PAME), 2013.
Pedrós-Alió, C., Potvin, M., and Lovejoy, C.: Diversity of planktonic microorganisms in the Arctic Ocean, Prog. Oceanogr., 139, 233–243, https://doi.org/10.1016/j.pocean.2015.07.009, 2015.
Poulin, M., Daugbjerg, N., Gradinger, R., Ilyash, L., Ratkova, T., and von Quillfeldt, C.: The pan-Arctic biodiversity of marine pelagic and sea-ice unicellular eukaryotes: a first-attempt assessment, Mar. Biodivers., 41, 13–28, https://doi.org/10.1007/s12526-010-0058-8, 2011.
Pućko, M., Dionne, K., and Michel, C.: Occurrence of toxin-producing marine algae in the Canadian Arctic and adjacent waters, Canadian Manuscript Report Fisheries And Aquatic Sciences, 3180, vii + 27, 2019.
Pućko, M., Rourke, W., Hussherr, R., Archambault, P., Eert, J., Majewski, A. R., Niemi, A., Reist, J., and Michel, C.: Phycotoxins in bivalves from the western Canadian Arctic: The first evidence of toxigenicity, Harmful Algae, 127, 102474, https://doi.org/10.1016/j.hal.2023.102474, 2023.
Rantanen, M., Karpechko, A. Y., Lipponen, A., Nordling, K., Hyvärinen, O., Ruosteenoja, K., Vihma, T., and Laaksonen, A.: The Arctic has warmed nearly four times faster than the globe since 1979, Commun. Earth Environ., 3, 1–10, https://doi.org/10.1038/s43247-022-00498-3, 2022.
R Core Team: R: A Language and Environment for Statistical Computing, R Foundation for Statistical Computing, Vienna, Austria [software], https://www.R-project.org/ (last access: 27 August 2024), 2024.
Reguera, B., Velo-Suárez, L., Raine, R., and Park, M. G.: Harmful Dinophysis species: A review, Harmful Algae, 14, 87–106, https://doi.org/10.1016/j.hal.2011.10.016, 2012.
Reid, P. C., Johns, D. G., Edwards, M., Starr, M., Poulin, M., and Snoeijs, P.: A biological consequence of reducing Arctic ice cover: arrival of the Pacific diatom Neodenticula seminae in the North Atlantic for the first time in 800 000 years, Global Change Biol., 13, 1910–1921, https://doi.org/10.1111/j.1365-2486.2007.01413.x, 2007.
Richardson, A. J., Walne, A. W., John, A. W. G., Jonas, T. D., Lindley, J. A., Sims, D. W., Stevens, D., and Witt, M.: Using continuous plankton recorder data, Prog. Oceanogr., 68, 27–74, https://doi.org/10.1016/j.pocean.2005.09.011, 2006.
Righetti, D., Vogt, M., Gruber, N., Psomas, A., and Zimmermann, N. E.: Global pattern of phytoplankton diversity driven by temperature and environmental variability, Sci. Adv., 5, eaau6253, https://doi.org/10.1126/sciadv.aau6253, 2019.
Righetti, D., Vogt, M., Zimmermann, N. E., Guiry, M. D., and Gruber, N.: PhytoBase: A global synthesis of open-ocean phytoplankton occurrences, Earth Syst. Sci. Data, 12, 907–933, https://doi.org/10.5194/essd-12-907-2020, 2020.
Rösel, A., Kaleschke, L., and Birnbaum, G.: Melt ponds on Arctic sea ice determined from MODIS satellite data using an artificial neural network, The Cryosphere, 6, 431–446, https://doi.org/10.5194/tc-6-431-2012, 2012.
Rudels, B. and Carmack, E.: Arctic Ocean Water Mass Structure and Circulation, Oceanography, 35, 52–65, https://doi.org/10.5670/oceanog.2022.116, 2022.
Schiffrine, N., Dhifallah, F., Dionne, K., Poulin, M., Lessard, S., Rochon, A., and Gosselin, M.: Microbial plankton occurrence database in the North American Arctic region: synthesis of recent diversity of potentially toxic and/or harmful algae – Code and Dataset, Zenodo [code and data set], https://doi.org/10.5281/zenodo.10498858, 2024.
Sournia, A.: Phytoplankton Manual, in: Monographs on oceanographic methodology, Unesco, Paris, 337 pp., ISBN 93-3-101572-9, 1978.
Thompson, G. G. and Withers, P. C.: Effect of species richness and relative abundance on the shape of the species accumulation curve, Austral Ecol., 28, 355–360, https://doi.org/10.1046/j.1442-9993.2003.01294.x, 2003.
Tillmann, U., Elbrächter, M., Krock, B., John, U., and Cembella, A.: Azadinium spinosum gen. et sp. nov. (Dinophyceae) identified as a primary producer of azaspiracid toxins, Eur. J. Phycol., 44, 63–79, https://doi.org/10.1080/09670260802578534, 2009.
Torres-Valdés, S., Tsubouchi, T., Bacon, S., Naveira-Garabato, A. C., Sanders, R., McLaughlin, F. A., Petrie, B., Kattner, G., Azetsu-Scott, K., and Whitledge, T. E.: Export of nutrients from the Arctic Ocean, J. Geophys. Res.-Oceans, 118, 1625–1644, https://doi.org/10.1002/jgrc.20063, 2013.
Tremblay, G., Belzile, C., Gosselin, M., Poulin, M., Roy, S., and Tremblay, J.-É.: Late summer phytoplankton distribution along a 3500 km transect in Canadian Arctic waters: strong numerical dominance by picoeukaryotes, Aquat. Microb. Ecol., 54, 55–70, https://doi.org/10.3354/ame01257, 2009.
Tremblay, J.-É., Anderson, L. G., Matrai, P., Coupel, P., Bélanger, S., Michel, C., and Reigstad, M.: Global and regional drivers of nutrient supply, primary production and CO2 drawdown in the changing Arctic Ocean, Prog. Oceanogr., 139, 171–196, https://doi.org/10.1016/j.pocean.2015.08.009, 2015.
Vihtakari, M.: ggOceanMaps: Plot Data on Oceanographic Maps using “ggplot2”, GitHub [code], https://mikkovihtakari.github.io/ggOceanMaps/ (last access: 27 August 2024), 2021.
Waleron, M., Waleron, K., Vincent, W. F., and Wilmotte, A.: Allochthonous inputs of riverine picocyanobacteria to coastal waters in the Arctic Ocean, FEMS Microbiol. Ecol., 59, 356–365, https://doi.org/10.1111/j.1574-6941.2006.00236.x, 2007.
Wassmann, P., Kosobokova, K. N., Slagstad, D., Drinkwater, K. F., Hopcroft, R. R., Moore, S. E., Ellingsen, I., Nelson, R. J., Carmack, E., Popova, E., and Berge, J.: The contiguous domains of Arctic Ocean advection: Trails of life and death, Prog. Oceanogr., 139, 42–65, https://doi.org/10.1016/j.pocean.2015.06.011, 2015.
Whittaker, R. H.: Evolution and Measurement of Species Diversity, TAXON, 21, 213–251, https://doi.org/10.2307/1218190, 1972.
Wickham, H., Averick, M., Bryan, J., Chang, W., McGowan, L. D., François, R., Grolemund, G., Hayes, A., Henry, L., Hester, J., Kuhn, M., Pedersen, T. L., Miller, E., Bache, S. M., Müller, K., Ooms, J., Robinson, D., Seidel, D. P., Spinu, V., Takahashi, K., Vaughan, D., Wilke, C., Woo, K., and Yutani, H.: Welcome to the tidyverse, J. Open Source Softw., 4, 1686, https://doi.org/10.21105/joss.01686, 2019.
Yamamoto-Kawai, M., McLaughlin, F. A., Carmack, E. C., Nishino, S., Shimada, K., and Kurita, N.: Surface freshening of the Canada Basin, 2003–2007: River runoff versus sea ice meltwater, J. Geophys. Res., 114, C00A05, https://doi.org/10.1029/2008JC005000, 2009.