the Creative Commons Attribution 4.0 License.
the Creative Commons Attribution 4.0 License.
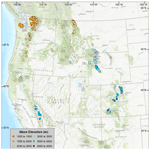
Inventory of glaciers and perennial snowfields of the conterminous USA
Bryce Glenn
Christopher Mcneil
This report summarizes an updated inventory of glaciers and perennial snowfields of the conterminous United States. The inventory is based on interpretation of mostly aerial imagery provided by the National Agricultural I magery Program, US Department of Agriculture, with some satellite imagery in places where aerial imagery was not suitable. The inventory includes all perennial snow and ice features ≥ 0.01 km2. Due to aerial survey schedules and seasonal snow cover, imageries acquired over a number of years were required. The earliest date is 2013 and the latest is 2020, but more than 73 % of the outlines were acquired from 2015 imagery. The inventory is compiled as shapefiles within a geographic information system that includes feature classification, area, and location. The inventory identified 1331 (366.52 ± 14.34 km2) glaciers, 1176 (31.01 ± 9.30 km2) perennial snowfields, and 35 (3.57 km2 ± no uncertainty) buried-ice features. The data including both the shapefiles and tabulated results are publicly available at https://doi.org/10.15760/geology-data.03 (Fountain and Glenn, 2022).
- Article
(16342 KB) - Full-text XML
- BibTeX
- EndNote
Glaciers are an important feature of the landscape for several reasons. Geologically, they modify the landscape through erosion and deposition (Alley et al., 2019; Benn and Evans, 2010). Although these processes are typically slow, sudden episodes can occur such as moraine failure due to fluvial erosion resulting in catastrophic debris flows (Beason et al., 2018; Chiarle et al., 2007; O'Connor et al., 2001). Hydrologically, glaciers can be viewed as frozen reservoirs of water that naturally regulate streamflow on seasonal to decadal timescales (Dussaillant et al., 2019; Fountain and Tangborn, 1985; Moore et al., 2009). Glacier runoff increases during warm periods and diminishes during cool, wet periods. Thus, glacier-populated watersheds have less seasonally variable runoff than ice-free watersheds. Also, glacier runoff cools stream temperatures in the driest and hottest part of the summer after seasonal snowpacks have vanished (Cadbury et al., 2008; Fellman et al., 2014). As glaciers shrink, they have less ability to buffer seasonal runoff variations, and watersheds become more susceptible to drought (Huss and Hock, 2018; Pritchard, 2019). Globally, the loss of perennial ice from the landscape is a major contributor to sea level rise (Meier, 1984; Parkes and Marzeion, 2018; Zemp et al., 2019).
Glacier inventories have been valuable for assessing glacier contribution to sea level change (Hock et al., 2009; Pfeffer et al., 2014) and for assessing regional hydrology (Moore et al., 2009; Yao et al., 2007). They also provide a baseline for quantifying glacier changes in the future. Updated glacier inventories have been compiled for many regions of the world (Andreassen et al., 2022; Bolch et al., 2010; Smiraglia et al., 2015; Sun et al., 2018). An exception has been the western United States (USA), defined here as those conterminous states west of the 100th meridian. The most recent inventory is Fountain et al. (2007, 2017) based on US Geological Survey (USGS) maps compiled over a 40-year period from the late 1940s to the 1980s. Despite a vigorous history of glacier studies (e.g., Armstrong, 1989; Rasmussen, 2009), glacial geology (e.g., Bowerman and Clark, 2011; Davis, 1988; Osborn et al., 2012), and regional inventories (e.g., DeVisser and Fountain, 2015; Fagre et al., 2017; Post et al., 1971), the glacier cover for the entire western USA has not been reevaluated.
The earliest scientific identification of glacier-populated regions in the western USA dates to King (1871) and, more comprehensively, to Russell (1898). The first summary of glacier-covered area for each state was Meier (1961). However, the data sources and methods used to compile the inventories are unknown. Denton (1975) summarized all known glacier studies in the western USA but did not tabulate glacier area. Krimmel (2002) updated Meier's study and provided total glacier area for the various mountain ranges by summarizing a variety of previous studies published over a more than 10-year time span. It is not clear whether the inventory is complete, and no data on individual glaciers are provided. Fountain et al. (2007, 2017) compiled the first comprehensive inventory of glaciers in the western USA. The data were derived from historical USGS 1:24 000 scale maps compiled over a 40-year period from the 1940s to the 1980s (Gesch et al., 2002; Usery et al., 2009). Because the USGS mapping was based on one-time aerial imagery, the misinterpretation of seasonal snow as perennial was extensive in some regions. The most current study, Selkowitz and Forster (2016), used Landsat satellite imagery compiled over a 4-year period, 2010–2014, and an automated detection scheme to define perennial snow and ice. However, these early automated schemes are known to misclassify debris-covered ice as ice-free landscape, underestimating glacier area (Earl and Gardner, 2016; Paul et al., 2007; Rabatel et al., 2017). Recent advances in automated detection have reduced these errors, suggesting a more promising future (Lu et al., 2022; Robson et al., 2020).
This paper presents the results of an updated and comprehensive inventory of glaciers and perennial snowfields of the western USA for the purpose of defining their current extent and to provide of baseline for estimating future changes. We summarize our methods, uncertainties, tabulated results, and data availability. The data referenced throughout the paper are publicly available at https://doi.org/10.15760/geology-data.03 (Fountain and Glenn, 2022).
2.1 Data sources, classification, digitizing, and completeness
The glaciers and perennial snowfields were initially located using a geographic information system (GIS) database from Fountain et al. (2007, 2017). New outlines were manually digitized from three sources of optical imagery. Most of the outlines were digitized from color digital orthographic aerial photographs available from the National Agricultural Imagery Program (NAIP), US Department of Agriculture, Farm Service Agency program (NAIP, 2017) (https://datagateway.nrcs.usda.gov/GDGHome_DirectDownLoad.aspx, last access: 2 December 2020). Since 2009, the imagery has been collected in cycles of 2 to 3 years. The aerial imagery was orthorectified using the inertial navigation system – GPS unit in the aircraft. Photo identifiable GPS-survey ground control points were then used to adjust the photo strip. Orthorectified strips, which had ≥ 30 % overlap with adjacent strips, were overlaid with each other and with ground control points to check accuracy. The image strips are then mosaicked together. The spatial resolution was ≤ 0.6 m with a horizontal accuracy of ≤ 6 m of photo-identifiable ground control points (NAIP, 2017). The NAIP imagery fits the historic USGS glacier outlines remarkably well. In a few cases, the NAIP imagery was not suitable due to seasonal snow, deep shadows, or image warping caused by orthophoto rectification; therefore, other sources were used including Maxar satellite imagery (Maxar Technologies, Inc.) with a spatial resolution of 0.5–1 m. For 21 perennial snowfields and three glaciers, we relied on the most recent snow-free imagery available in Google Earth (Google, Inc.) (resolution ∼ 1 m), because no other imagery was suitable. The outlines were digitized in Google Earth and exported to ArcMap (Esri, Inc.).
We manually identified all glaciers, ice patches, and perennial snowfields. Glaciers are defined as perennial snow and ice that moves (Cogley et al., 2011). A feature was considered perennial if it was present on the original 1:24 000 USGS topographic maps and present on all Google Earth imagery. Movement was identified by the presence of crevasses. Perennial snowfields and ice patches do not exhibit movement, as indicated by a lack of crevasses observed in the imagery. We do not distinguish between snowfields and ice patches and refer to both as perennial snowfields.
Contiguous glacier cover, most commonly on volcanoes, was separated into individual glaciers if they had unique names as indicated on the USGS maps. The orientation of crevasse patterns was used to define flow divides. In the absence of these patterns, shaded relief maps from digital elevation models were used. These models were derived from aerial lidar data, flown under contract to the USGS (Bard, 2017a, b, 2019; Robinson, 2014) or the Oregon Department of Geology and Mineral Industries (DOGAMI, 2011).
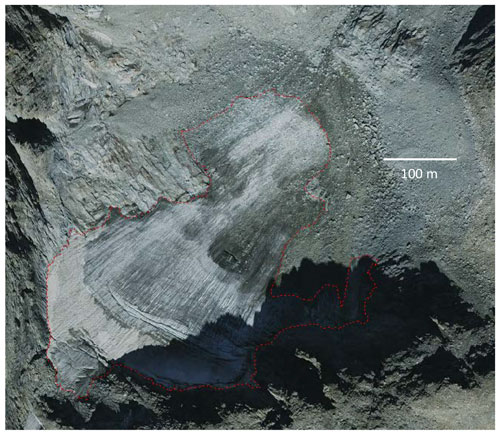
Figure 1An example of a glacier seemingly melting into the talus surrounding the terminus (upper right). The light red dashed line is the digitized perimeter. The glacier is flowing from the lower left-hand corner to the upper right-hand corner. The glacier is located in the Wind River Range, WY (inventory ID, INV_ID, E618081N4774579), and the base image is from the National Agricultural Image Program, taken in 2015.
We encountered a number of challenges to our classification and delineation of the glaciers and perennial snowfields. Although crevasses were used to define movement, in a few cases it appeared that they penetrated through the feature to the bedrock underneath, suggesting a mechanical breakup. In these cases, the feature was classified as a snowfield. For some glaciers, rock debris-cover made defining the glacier outline difficult. Fortunately, this problem was largely confined to the glaciers mantling the volcanoes of the Cascade Range. We relied on local knowledge to help define some boundaries and independent digitization efforts by the authors and others to provide an uncertainty as explained below. In the high alpine regions of California, Colorado, and Wyoming, the termini of some glaciers were hard to define. Rather than abruptly terminating, the ice seems to thin and smoothly transitions into the surrounding rock talus (Fig. 1). It was unclear whether a thin debris layer blanketed the ice or cobbles and boulders protruded through the thin ice. The boundary was mapped along the edge of identifiable ice.
In a few situations, we found it difficult to distinguish glaciers from rock glaciers (Brardinoni et al., 2019). A rock glacier is a mass of rock debris in a matrix of ice that flows (Cogley et al., 2011). They can be difficult to distinguish from a debris-covered glacier, one that has extensive rock debris over the ablation zone, that lower part of a glacier with exposed ice in late summer. We adopted the following topographic classification. If the slope of the apparent ice patch/snowfield was similar to the slope of the rock glacier, then we considered it part of the rock glacier (Fig. 2a). On the other hand, if a topographic depression separates the apparent glacier/snowfield from the start of a rock glacier, then it was considered an independent feature (Fig. 2b). This latter case is similar to the “glacier forefield-connected” rock glacier as described by RGIK (2022).
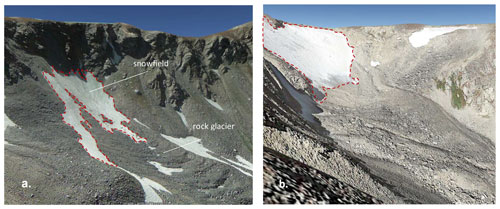
Figure 2Examples of glacier versus rock glacier identification. (a) An example of a snowfield that is considered part of the rock glacier. Location, Colorado Front Range; 40.827477∘ N, 106.657400∘ W. The light red dashed line is the snowfield/glacier perimeter. The image is from © Google Earth, September 2014; (b) Tyndall Glacier in the Colorado Front Range; 40.305291∘ N, 105.689602∘ W, with a rock glacier slightly down the valley. Image is from © Google Earth, September 2016.
In a number of situations, we observed buried ice adjacent to a glacier (Fig. 3). Here we use the term “buried ice” to mean dead ice formerly part of a flowing glacier and not the permafrost context of ice embedded within or on top of perennially frozen ground. The rocky surface texture of the buried ice was hummocky and very different from surrounding bedrock and adjacent ice and not a moraine. Occasionally a crack in the surface revealed subsurface ice. The feature appeared to be non-moving (dead) ice that is covered by debris similar to some of the ice-debris complexes described by Bolch et al. (2019). We decided to include these features as a separate classification, buried ice, because their size was large relative to the glacier; they were probably once part of the glacier and may be important local sources of meltwater for streamflow.
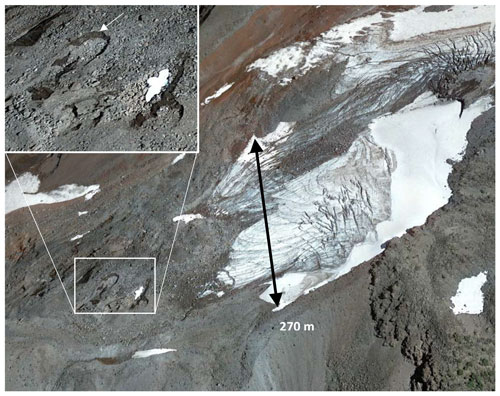
Figure 3Lost Creek Glacier, South Sister, Oregon. Note the buried ice and lack of crevasses to the left of the grey-blue ice, suggesting ice that is no longer moving and therefore not part of the dynamic glacier. The white box surrounds an area that has collapsed due to subsurface melt. The inset enlargement shows a cliff edge of exposed dirty ice (white arrow in upper left) indicated by a darker color suggesting wet sediment and a finer texture than the surface debris. The black arrow shows the width of the cleaner ice for scale. Image is from © Google Earth, 9 August 2021.
The glacier and perennial snowfield outlines were digitized using ArcMap (Esri, Inc.), a geographic information system, at scales varying from 1:300 to 1:2000, depending on image quality and complexity. We used the native projection of the image, North American Datum of 1983 (NAD83) for the NAIP, and World Geodetic System 1984 (WGS84) for Maxar and Google Earth. When Maxar or Google Earth imagery was used, final outlines were projected onto the NAD83 coordinate system. Google Earth was often used as an additional aid in interpretation because of its tilt and rotation features that yielded oblique perspectives. Retaining only those outlines ≥ 0.01 km2, each was checked independently by the two senior authors of this report and in some cases by a third collaborator in order to reduce bias (Leigh et al., 2019). If an outline was revised, then it was returned to its original author for review and correction, and the process iterated until all parties agreed.
Our initial inventory was then compared sequentially to two other independent inventories to test for errors of omission or commission. The first comparison was to the Selkowitz and Forster (2016) inventory (SFI). However, to compare the inventories we had to first reconcile the differences in methods. Buried-ice features were eliminated from our inventory because the SFI did not map buried ice. The SFI was filtered to only include features ≥ 0.01 km2 to match our minimum area threshold; a small number of features located in Canada were removed; and a few misclassifications of ponds, lakes, and dry lake beds as glaciers were removed. Notably, the SFI did not split contiguous ice masses, such as glacier-covered volcanoes, into individual glaciers; consequently, we do not expect the number of features in the SFI and our inventory to match. Once the two inventories were reconciled, those glaciers and perennial snowfields unique to one inventory were examined for inclusion in a revised inventory. Features selected from the SFI were digitized using the same imagery we used for our inventory.
The revised inventory was then compared to the 2016 National Land Cover Database (NLCD) (Dewitz, 2019), which did not map glaciers and perennial snowfields per se but mapped the distribution of perennial snow and ice (Jin et al., 2019; Wickham et al., 2021). However, the NLCD used a small number of recent images to assess a “perennial” presence; therefore, significant errors of commission are expected. Also, the landscape class of snow and ice received less attention than other classes (e.g., agriculture) such that the timing of imagery acquisition may be earlier in the summer than optimal, and misclassification of clouds as snow and ice may be present (Collin Homer and Jon Dewitz, USGS, personal communication, email December 2015). The NLCD inventory was compared to the revised inventory and, as before, the features unique to one inventory were examined for inclusion. Those features selected from the NLCD for inclusion were digitized using the same imagery we used for our inventory.
2.2 Uncertainty
Three main sources of uncertainty in the glacier outlines are georeferencing, digitization, and interpretation (DeVisser and Fountain, 2015; Sitts et al., 2010). We found the georeferencing error to be very small. In any case, the precise location of the outline does not affect its area. Also, the digitized points are highly correlated such that no deviations from the true outline are caused by georeferencing. Digitizing error is relatively small, 1 %, with good imagery and crisp contrast between the glacier and ice-free surroundings (DeVisser and Fountain, 2015; Hoffman et al., 2007). The largest uncertainty is interpretation error caused by poor imagery, shadow, debris cover, and seasonal snow patches. This uncertainty was calculated in different ways according to the situation. If the outline was digitized a second (or third) time due to different interpretations by the authors or collaborators, the uncertainty is one-half the absolute difference of that between the largest and smallest digitized areas (the range) divided by the final area and expressed as a percentage. For the relatively few glaciers where a small section of perimeter was masked by deep shadow, seasonal snow patches, rock debris, or poor imagery, a higher uncertainty was assigned by visually estimating the area in question and dividing by the total possible area. In a few cases, the location of a flow divide between glaciers was not clear, so a 5 % error was assigned. This was calculated from the area difference in several test cases where multiple possible flow divides were digitized. For perennial snowfields, the smaller patch of perennial snow is often covered by seasonal snow, which varies greatly from year to year. We measured the area of a number of snowfields over time using late summer historic imagery in Google Earth. Results showed that the variations in snowfield area could be as much as 30 %. We assigned this somewhat arbitrary uncertainty in order to note snowfield presence and location, but we preclude them from area change calculations because area differences are typically smaller than the assigned uncertainty.
Our initial inventory identified 2267 glaciers and perennial snowfields totaling 391.95 km2. About 70 % (1576) overlapped the features in the SFI. After examining all features unique to each inventory, we revised our inventory to include 2373 (394.99 km2) glaciers and perennial snowfields. Comparing the revised inventory to the 2016 NLCD resulted in adding another 134 (2.53 km2) features, which included 12 (0.38 km2) glaciers. The final inventory includes 2542 features composed of 1331 (366.52 km2) glaciers, 1176 (31.01 km2) perennial snowfields, and 35 (3.57 km2) buried-ice deposits (Table 1; Fig. 4). Most glaciers and perennial snowfields, 1554 (62 %), were outlined using the 2015 NAIP imagery, with the remainder outlined using mostly NAIP imagery from 2013 to 2020.
Table 1The summary of the glacier inventory for the American west, exclusive of Alaska. “Number” is the total number of features within each classification (class), “Max area” is the largest area of the feature within that class, and “Mean area” is the average area. Note that the uncertainty of “buried ice” is unknown.
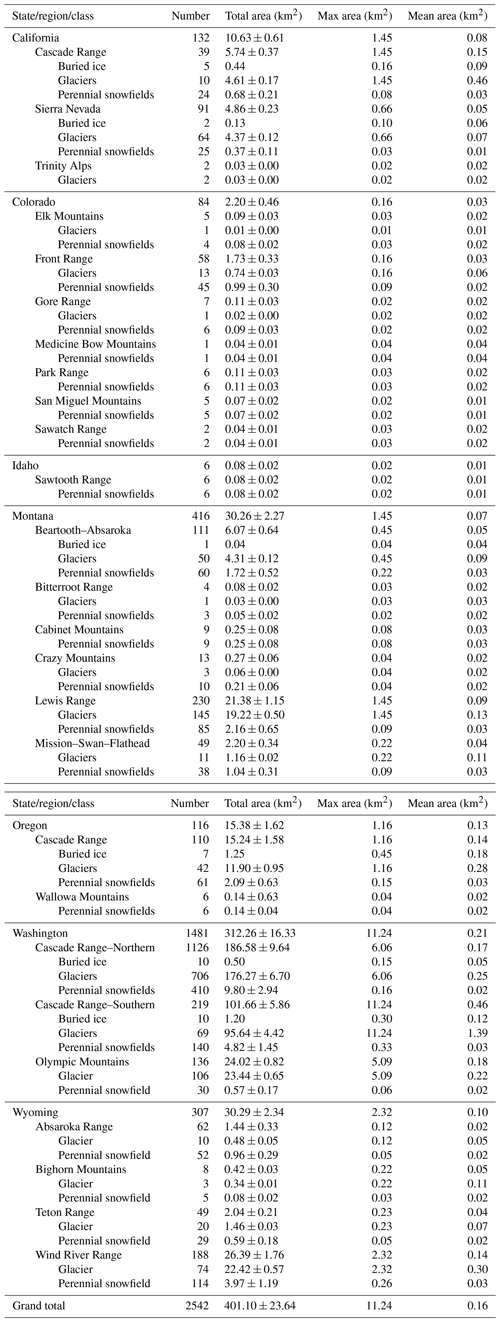
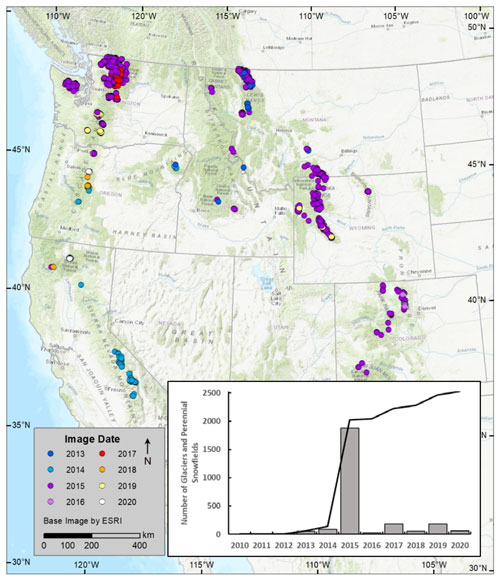
Figure 4The spatial distribution and number of glaciers and perennial snowfields, greater than 0.01 km2, in the western United States. Colors indicate the date of aerial and satellite imagery used to outline the features. The line is the cumulative total. The base imagery is from Esri, Inc. The inset is a bar graph and cumulative sum of the number of glaciers and perennial snowfields digitized in each image date.
Before summarizing the inventory data, we give a note about the content in Appendix A. It summarizes the officially named glaciers that we regard as snowfields or missing; labeling issues found in the USGS Geographic Names Information System, the official agency responsible for hosting the names and locations of landscape features; and detailed notes, organized by US state, on the specific imagery used and challenges encountered digitizing glacier and snowfield outlines.
The glaciers and perennial snowfields are generally small, averaging 0.28 and 0.03 km2, respectively. Like glaciers elsewhere in the Northern Hemisphere, most glaciers face north to east (Evans, 2006; Fountain et al., 2017; Schiefer et al., 2007). The distribution of glacier area is skewed towards smaller ice masses (Fig. 5a). The state of Washington in the Pacific Northwest has the largest number of glaciers, ice area, and the largest glacier (11.24 km2 Emmons Glacier) of any of the other states (Table 1). Indeed, the glacier cover on Mount Rainier alone (77.37 km2) is greater than the total sum in all the other states (71.16 km2). The elevation distribution of glacier-covered area is bimodal with maxima at 2400 and 3650 m (Fig. 5b). The spatial distribution of elevations shows a regional climate control with the lowest glaciers and perennial snowfields in the maritime climate of the Pacific Northwest of Washington, Oregon, northern California, and western Montana and the highest elevations located in the continental climate of central California, Colorado, Wyoming, and southern Montana (Fig. 6).
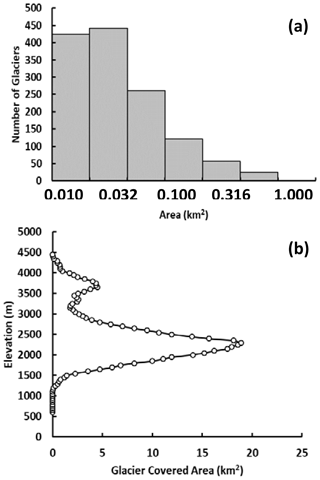
Figure 5The area and elevation distribution of glaciers in the western USA. (a) Histogram showing the number of glaciers as a function of area. The x-axis intervals are log intervals; (b) elevation distribution of glacier-covered area.
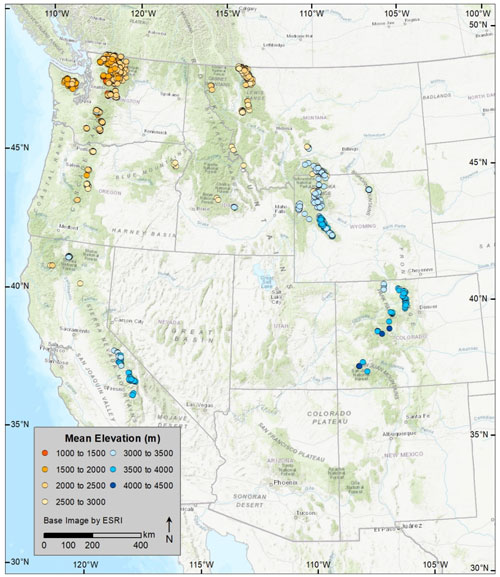
Figure 6Elevation distribution of glaciers and perennial snowfields across the western USA; base imagery from Esri, Inc.
The final inventory conflicts with the current database of the Geographic Names Information System (https://www.usgs.gov/us-board-on-geographic-names/domestic-names, last access: 20 December 2022). The inventory excludes 52 officially named glaciers because 2 have disappeared, 25 were classified as perennial snowfields, the areas of 18 were less than 0.01 km2, and 7 were considered rock glaciers (Appendix A, Table A1). In some cases, a named glacier or snowfield had split into multiple pieces since the original USGS mapping; all pieces were assigned the same name in the inventory (Appendix A, Table A2). Several labels that identify the name of the glacier are not clearly associated with a specific glacier, and these are listed in Table A3.
The advent of relatively frequent high-resolution (≤ 1 m) optical, aerial, and satellite imagery available at little or no cost has made compiling and updating glacier inventories a realistic opportunity. Finding suitable imagery spanning only a few years apart provides a near-snapshot of glacier cover. And the advent of GIS software made digitizing, summarizing, and interrogating digital outlines practical. This contrasts strongly with mapping efforts only a few decades ago when aerial-only photographic surveys required decades to cover the western USA, and georectification, outlining, and map production were slow (Gesch et al., 2002).
We had used the Fountain et al. (2017) historic inventory as a template to locate and update the perimeters of all the glaciers and perennial snowfields. Considering that the inventory was derived from the US Geological Survey 1:24 000 maps, a result of a national effort to remap the entire country at a higher resolution, we were surprised that 240 features (∼ 10 %) were missed. These missing features were revealed after comparison with two other independently derived inventories. We had a similar experience in a prior study when comparing two independently derived glacier inventories. Together, they suggest that independent efforts are important when compiling a comprehensive inventory.
Multiple checks more accurately define glacier perimeters (Leigh et al., 2019). Different investigators may make different decisions about glacier boundaries, and results can differ particularly in debris-covered conditions or along flow divides (Paul et al., 2013). When they agree, it provides some confidence of the interpretation accuracy, and when they disagree it provides input for estimating interpretation error.
The total area of glaciers in the western USA, 367 km2, is a little smaller than that in Austria, 415 km2 (Fischer et al., 2015). Like glacier-populated regions elsewhere, the distribution of glacier area is skewed towards smaller glaciers (e.g., Linsbauer et al., 2012; Mishra et al., 2023; Zalazar et al., 2020). The uncertainty in glacier area is also similar with an overall 5 % uncertainty for the total area. This compares favorably with those reported in the literature: 3.3 % for a set of 15 glaciers (Paul et al., 2020), 4 % for 7 glaciers (Zalazar et al., 2020), and 2.3 % for 15 glaciers (Linsbauer et al., 2021). Our assessment method differs from those cited here in that we estimate the uncertainty for each individual glacier rather than upscaling the uncertainty calculated for a small subsample.
The data are available in three formats. The geospatial data and attribute tables are available in the shapefile (Esri) format and in an open-source GeoJSON format. The attribute table is also available as a comma separated values (CSV) file. These data products can be obtained from https://doi.org/10.15760/geology-data.03 (Fountain and Glenn, 2022) and from the Global Land Ice Measurements from Space website (http://glims.colorado.edu/glacierdata/, last access: 8 June 2023). Maxar imagery was accessed through the USGS and NGA NEXTVIEW license. The Maxar imagery has limited availability, owing to restrictions (proprietary interest). Contact cmcneil@usgs.gov for more information.
We have compiled a new and comprehensive inventory of glaciers and perennial snowfields in the western USA from aerial and satellite imagery. Results show that 2542 features are currently present and include 1331 (366.52 km2) glaciers, 1176 (31.01 km2) perennial snowfields, and 35 (3.57 km2) buried-ice deposits. Most of the data were acquired from the 2015 NAIP imagery, with the remainder from NAIP imagery and a few satellite images acquired over the period of 2013 to 2020. The state of Washington has the greatest number and area of glaciers and perennial snowfields. This product updates an older inventory based on USGS 1:24 000 maps compiled in the middle-to-late 1900s. The new inventory is a significant improvement in accuracy, because the archive of historical imagery in Google Earth greatly aided our efforts to classify glaciers versus perennial snowfields. Finally, this new inventory provides a baseline for assessing glacier change in the conterminous USA.
A1 Missing glaciers
Table A1List of officially named glaciers not classified as glaciers and excluded from the final inventory. Names come from the Geographic Names Information System (https://www.usgs.gov/tools/geographic-names-information-system-gnis, last access: November 2022). The “Reason” column lists why the named glacier is no longer considered a glacier in our inventory.
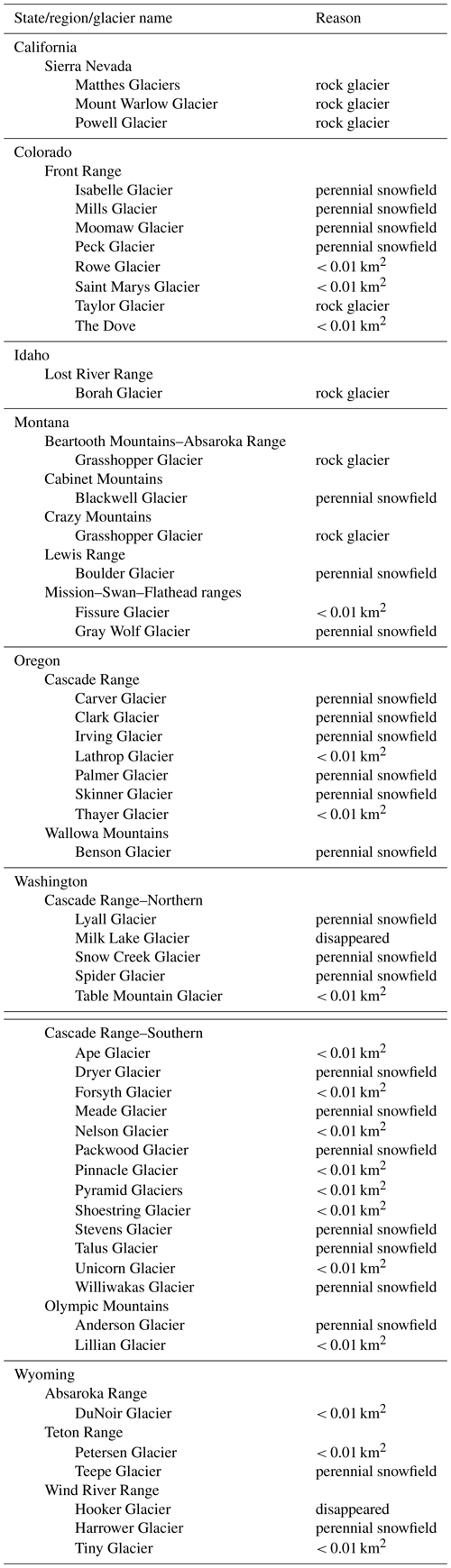
A2 Glaciers that have split into multiple pieces and current errors in glacier label names
Table A2List of named glaciers that have split into multiple pieces. Names come from the Geographic Names Information System (https://www.usgs.gov/tools/geographic-names-information-system-gnis, last access: November 2022). “Count” refers to the number of pieces in the updated inventory. “Classes” is the classification of the pieces: glacier, perennial snowfield, buried-ice, or a combination.
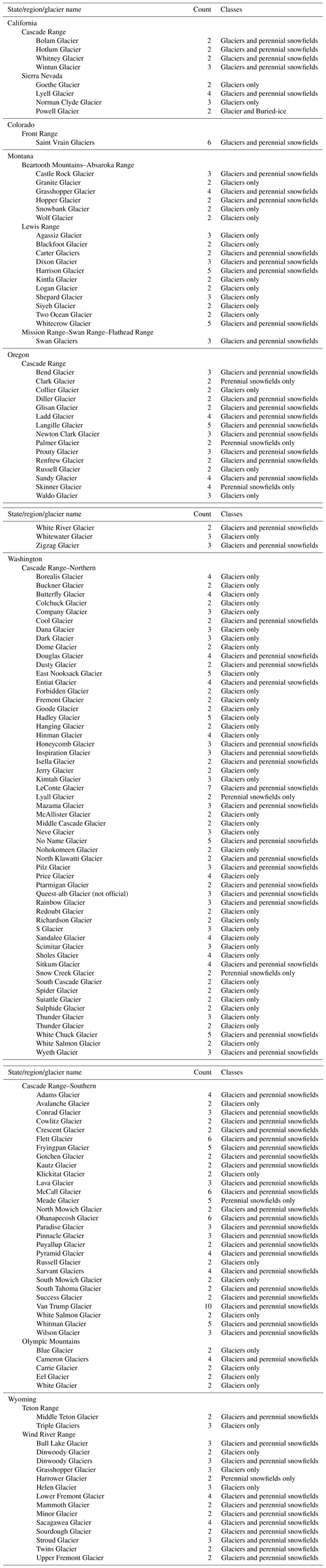
A3 Labeling errors in the US Geographic Names Information System
Table A3List of officially named glaciers where we identified an issue with the glacier name on the 1:24 000 US Geological Survey topographical maps (Fountain et al., 2017). Names come from the Geographic Names Information System (https://www.usgs.gov/tools/geographic-names-information-system-gnis, last access: November 2022). The “Issue” column lists the type of issue identified, “Not labeled” indicates the feature was present but not labeled, “Misidentified” indicates the wrong feature was labeled, and “Label unclear” means the location of the label is not clearly associated with a specific glacier.
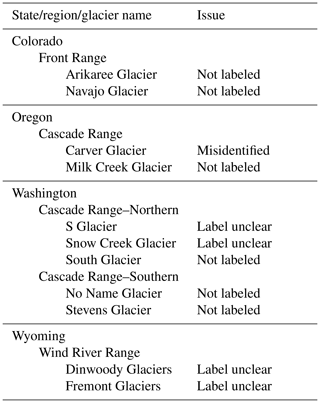
A4 Notes on imagery and interpretation challenges by state
This appendix, organized by US state and then by mountain range, summarizes the specific imagery used and challenges encountered in feature identification and digitization. The Selkowitz and Forster (2016) inventory is referred to as the SFI, and the National Land Cover Database inventory (Dewitz, 2019) is referred to as the NLCD.
A4.1 California
Imagery and DEMs used are listed in Tables A4–A6.
Table A4List of the NAIP imagery used for outlining glaciers and perennial snowfields in California. “Date” is the start and end dates for flights covering the glaciated portions of the NAIP imagery. In some cases, flights were completed in a single day.
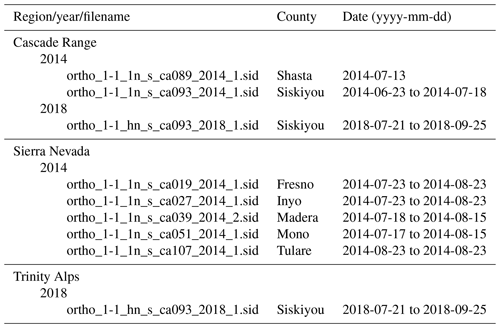
Table A5List of dates of the Maxar imagery used for outlining glaciers and perennial snowfields in California.
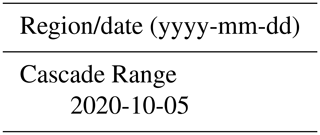
Cascade Range
Mount Shasta
The 2020 black-and-white Maxar imagery was most useful because of the minimal seasonal snow cover. The 2018 NAIP imagery was helpful in situations where the 2020 imagery was obscured by shadow, distortion, or misaligned and when color was needed to improve interpretation. The 2010 lidar DEM (Robinson, 2014; Table A4) was used to create a multidirectional hillshade to improve perspective and interpretation (Fig. A1).
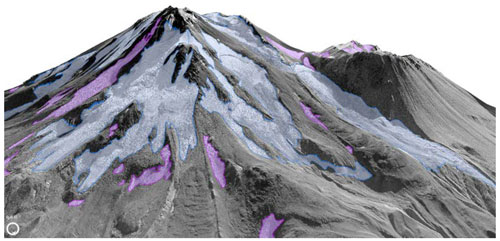
Figure A1Mt. Shasta glaciers in bluish white; perennial snowfields/ice patches in lavender draped over a 3D rendering created from 2010 lidar (Robinson, 2014).
The rock debris on the termini of most glaciers and on some of the upper parts of the glaciers was challenging to interpret. It was hard to determine whether ice was present under the debris and whether that ice is part of the active glacier. Spatial patterns of debris, debris contrasts, and melt streams flowing from the debris were used to estimate the glacier boundaries.
Sierra Nevada
The 2014 NAIP imagery was the best imagery due to low snow cover. In some cases, features were difficult to outline because of shadow or image quality. In these cases, 2013/2012 Google Earth imagery was used. Some glaciers were reclassified as rock glaciers by Trcka (2020). These were re-examined, and where we agreed they were removed from the initial glacier inventory. Defining whether the feature was a glacier or rock glacier was often difficult; see the Colorado section for more discussion.
Trinity Alps
The 2018 imagery was the best for the least snow cover. Justin Garwood (Garwood et al., 2020) provided outlines for two glaciers: Grizzly and Salmon. The area of the most recent outline of the Salmon Glacier was < 0.01 km2 and was not included in this inventory. By 2018 all of the other features mapped by the USGS (Fountain et al., 2017) were less than 0.01 km2 or had disappeared. An additional feature was added based on the 2016 NLCD (Jin et al., 2019).
A4.2 Colorado
The 2015 NAIP was generally free of seasonal snow. Where it persisted at the termini of a few glaciers; images for the same year in Google Earth aided perimeter interpretation. The imagery used is listed in Table A7.
Elk Mountains
No features were mapped in the Elk Mountains by the USGS (Fountain et al., 2017). One glacier and four perennial snowfields were added from the SFI.
Front Range
The most recent inventory for the Front Range was Hoffman et al. (2007), which used aerial photographs to map the 2001 extent of glaciers. Many features in the Front Range are difficult to classify. The issue is the difference between a glacier or perennial snowfield and a rock glacier. Those that are part of the rock glacier are deleted from the glacier inventory. Those that seem to be separate from rock glaciers are retained. This is a judgment call. From a hydrological point of view, if a snow-ice patch that is part of a rock glacier was counted separately from a rock glacier, then it is double counting a water feature.
A4.3 Idaho
The image quality was generally snow free. Of the glaciers mapped by the USGS (Fountain et al., 2017), only two remain and are classified as perennial snowfields. The Borah Glacier was officially named in 2021 (US Board on Geographic Names), but it is < 0.01 km2 and is not included in the inventory. Table A8 lists the imagery used.
A4.4 Montana
Image quality varied between mountain ranges due to differences in snow cover. Tables A9 and A10 list the imagery used.
Table A9List of the NAIP imagery used for outlining glaciers and perennial snowfields in Montana. “Date” is the start and end dates for flights covering the glaciated portions of the NAIP imagery. In some cases, flights were completed in a single day.
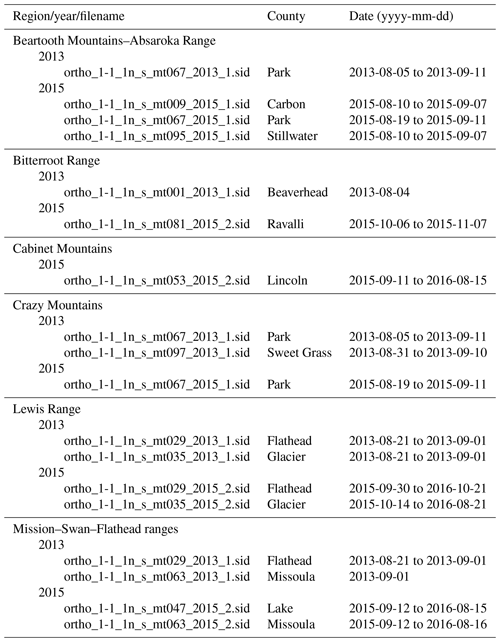
Beartooth Mountains–Absaroka Range
The 2015 NAIP imagery was the best overall imagery due to the least snow, but Google Earth was occasionally used as well. Google Earth had imagery dated to 11 September 2015, often with less seasonal snow than the NAIP imagery. To counter any mismatch in projection, outlines digitized in Google Earth were imported to ArcGIS and projected to match the NAIP projection.
Bitterroot Range
No features were mapped in the Bitterroot Range by the USGS (Fountain et al., 2017). One glacier and three perennial snowfields were added based on the NLCD.
Cabinet Range
The USGS mapped four features ≥ 0.01 km2 (Fountain et al., 2017). On inspection of the 2015 data, only one was ≥ 0.01 km2. Seven glaciers and perennial snowfields were added; five were identified in our initial inventory, and the other two were identified by the SFI and NLCD, respectively. All were less than 0.05 km2.
Crazy Mountains
The 2013 NAIP imagery was the best imagery available and included limited seasonal snow. The 2019 Maxar imagery had too much seasonal snow.
Lewis Range (Glacier National Park)
The most recent published glacier inventory is a 2015 USGS inventory (Fagre et al., 2017). That inventory outlined the main-body of named glaciers using 2015 Maxar imagery. We digitized the outlines of all glaciers and perennial snowfields using 2015 Maxar imagery where available. Elsewhere, the 2015 and 2013 NAIP imageries were used; both years had lots of seasonal snow cover. Two major glaciers, Blackfoot (Fig. A2) and Harrison (Fig. A3) glaciers, separated into pieces as they retreated since they were originally mapped by the USGS (Fountain et al., 2007).
Madison Range
The 2013 NAIP imagery was the only imagery used due to extensive snow in the other years. No glaciers or perennial snowfields were found. Of the two features ≥ 0.01 km2 mapped by the USGS (Fountain et al., 2017), the 2013 imagery showed that one feature is a rock glacier and the other was less than 0.01 km2.
Mission–Swan–Flathead ranges
Based on the least snow cover, the 2013 NAIP was better in the Mission and Flathead ranges, and the 2015 NAIP was better in the Swan Range. No glaciers or perennial snowfields remain in the Flathead Range.
A4.5 Oregon
Tables A11–A13 list the imagery and DEM used.
Table A11List of the NAIP imagery used for outlining glaciers and perennial snowfields in Oregon. “Date” is the start and end dates for flights covering the glaciated portions of the NAIP imagery. In some cases, flights were completed in a single day.
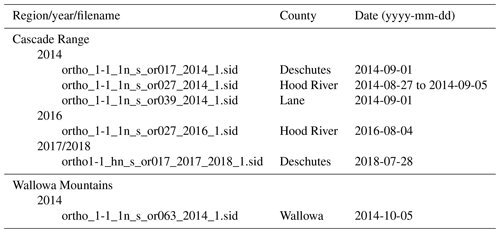
Table A12List of dates of the Maxar imagery used for outlining glaciers and perennial snowfields in Oregon.
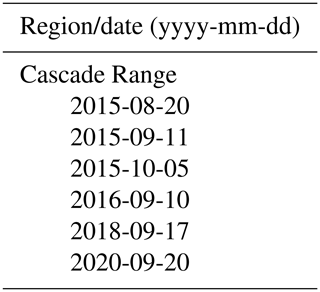
Cascade Range
Seasonal snow cover was commonly present when this range was imaged by any of the sensors, making it difficult to find suitable imagery.
Mount Hood
The most recent glacier outlines for Mt. Hood were based on 2015 and 2016 Maxar color imagery with interpretation aid using Google Earth. Due to seasonal snow, some professional judgment was required in places.
Mount Jefferson
The 2018 NAIP imagery had extensive seasonal snow and was generally only useful near the termini of some glaciers. We used 2018 Maxar imagery that showed little seasonal snow, but it was a little cloudy and masked a bit of Whitewater Glacier. We also used Google Earth to help interpret some of the features.
Three Sisters
Maxar 2018 imagery was used, but the image was stretching along the feature's headwall; for that segment of the outline, the 2018 NAIP imagery was used. Two versions of the Maxar imagery for the same day are available, one color and one black and white. Color was georectified but suffered stretching along some headwalls. A light early-season snowfall occurred before the Maxar image was acquired, and the snow accumulated in some places just enough to obscure the surface. So, the glacier or snow patch outline was the minimum of the two images with occasional interpolation across the snowy surface to the nearest glacier edge.
Mount Thielsen
The Lathrop Glacier was named in 1981. At the time of the USGS mapping and now it is < 0.01 km2 and not counted as part of the inventory. Furthermore, Lathrop Glacier has been known to disappear in some years and therefore fails the definition of a glacier.
Wallowa Mountains
No NAIP imagery was useful, and Maxar did not image this region. We used the 30 August 2013 image from Google Earth, which was excellent with little snow. Features were digitized in Google Earth and then imported into ArcGIS. Because we used NAIP as the base imagery, we revised the outline from the projection in WGS84 (Google Earth) to NAD83 Universal Transverse Mercator (UTM) zone 11 (NAIP).
A4.6 Washington
The 2015 NAIP imagery was typically excellent with little snow cover, whereas the 2017 NAIP imagery had more snow and the 2019 imagery had lots of snow. For most outlines, 2015 NAIP imagery was used. In some places, the 2017 NAIP imagery had less snow and was used instead. Maxar imagery was of limited use and often was not better than the 2015 or 2017 NAIP data. Tables A14–A16 list the imagery and DEMs used.
Table A14List of NAIP imagery used for outlining glaciers and perennial snowfields in Washington. “Date” is the start and end dates for flights covering the glaciated portions of the NAIP imagery. In some cases, flights were completed in a single day. For 2006, the inspection date was used, since the start and end dates were not provided.
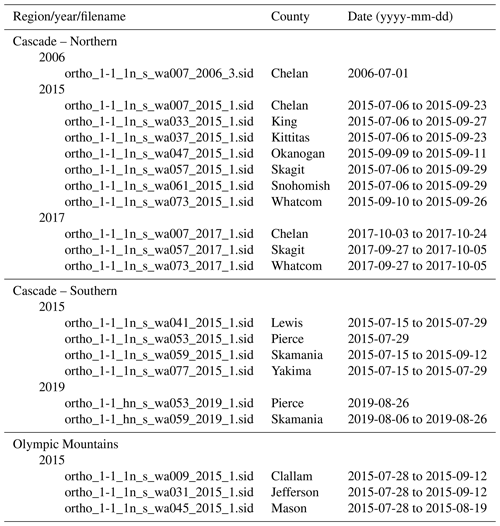
Table A15List of dates of the Maxar imagery used for outlining glaciers and perennial snowfields in Washington.
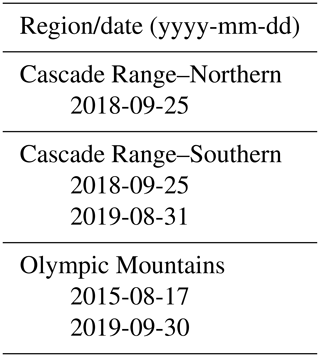
Cascade – Northern
The glaciers and perennial snowfields were previously inventoried by Dick (2013).
Mount Baker
The 2015 NAIP imagery was the best and had little seasonal snow. Google Earth 2009 and 2019 imagery were used to help interpretation. A multidirectional hillshade and 3 m contour lines derived from a lidar DEM (Bard, 2017a) were used to help define flow divides between glaciers, debris covered-ice, and buried ice. There are notable differences between the NAIP imagery and DEM data, particularly in steep terrain, areas of dark shadow, and debris-covered areas. The DEM helped correct these positional errors and had the benefit of supplying more information on surface texture.
Several buried-ice features were identified. The ice appeared to have decoupled from the active glacier. In a few cases, debris-covered ice is included in the glacier outline because the ice appears to be directly connected to the glacier, and there was evidence of movement.
Dragontail Peak
The USGS Geographic Names Information Service (GNIS) locates Snow Creek glacier at a point on the edge of the southeast glacier (Fountain et al., 2007). In the 2015 imagery, the point is on bedrock, making it unclear which glacier the GNIS is naming. The USGS identifies both glaciers as Snow Creek glacier. We labeled both glaciers as the Snow Creek glacier.
Glacier Peak
For the Glacier Peak region, a multidirectional hillshade and 3 m contour lines derived from a 2015 lidar DEM (Bard, 2017b) were used as a guide to define flow divides.
Hurry-up Peak
The point location of the South Glacier provided by the GNIS is over bedrock. We assume the point refers to the glacier located ∼ 150 m to the north of the point.
Cascade – Southern
Goat Rocks
Imagery from 2015 was best but had more snow than desired. Too much snow was present in 2017, but some ice was exposed. The 2019 imagery was too snowy for glacier digitization.
The outlines are almost entirely based on 2015 imagery, with a few based on 2017 imagery, where needed. We used 2009 NAIP imagery to help define the headwalls at the Conrad, McCall, and Packwood glaciers. Heard (2000) previously mapped the glacier perimeters. The maximum extent of the seasonal snow covering the terminal regions was not digitized. Typically, the glaciers and perennial snowfields were digitized at scales of 1:600 to 1:800. Note that the narrow arms of the snowfields were not typically digitized, knowing that they would probably disappear a few days to a week from the time of imagery.
Mount Adams
No suitable NAIP imagery was found; instead, 2019 Maxar imagery was used. In addition to the Maxar imagery, a multidirectional hillshade and 3 m contour lines derived from a 2016 lidar DEM (Bard, 2019) were used as a guide when delineating flow divides. Occasionally, 2009 Google Earth imagery was also useful. Extensive snow covered the mountain when the 2016 lidar was flown, masking some of the glacier termini. However, the DEM was helpful in correcting the imagery where it was poorly aligned with the terrain.
Multiple buried-ice features were identified near the termini of several glaciers where ice appeared to have decoupled from the main active glacier. Large areas below the glaciers (Mazama, Adams, and Pinnacle) likely have debris-covered ice. We focused on the features which were likely to contain ice based on meltwater streams exiting near the features and hummocky terrain which appeared to indicate melt. Ground-based images taken between 2014 and 2018 helped decision-making. The images were particularly helpful in identifying a debris-covered ice cliff at Adams Glacier.
Mount Rainier
In general, the 2019 NAIP and the Maxar (25 September 2018) imageries were used for the outlines. Although the GNIS includes the Nisqually Icefall as a separate feature, we included the icefall as part of the Nisqually Glacier (Fig. A4).
Mount St Helens
We used a GIS layer of geological mapping units that included snow and ice from the USGS (David Sherrod, USGS, personal communication, 2021) to help guide our search. The Crater Glacier (INV_ID E562842N5115499) was heavily debris covered and obscured by shadow in some areas.
Olympic Mountains
A 2015 inventory of the region was compiled because more recent imagery (NAIP and Maxar) was not useful due to seasonal snow. Our updated inventory differs from that published in Fountain et al. (2017) in two ways. First, they outlined and grouped the glaciers and perennial snowfields according to watershed rather than individual glacier. Their goal was to estimate glacier change relative to a previous study by Spicer (1986) and had to follow Spicer's approach. Second, all outlines were rechecked and compared to SFI and the NLCD, resulting in minor changes.
A4.7 Wyoming
Wind River Range
Tables A17 and A18 list the imagery used. The 2015 NAIP imagery had little snow in contrast to the 2019 imagery. Shadows are common in the 2015 imagery and can be very dark. Occasionally, the 2019 imagery was used to define the glacier–bedrock headwall boundary. The 2019 Maxar imagery was essentially identical to the NAIP imagery and not as useful as it was black and white. Imageries from 2017 and 2018 were a bit too snowy around the glacier margin to be useful. The 6 September 2018 Maxar imagery covered the entire range, with some clouds.
Table A17List of NAIP imagery used for outlining glaciers and perennial snowfields in Wyoming. “Date” is the start and end dates for flights covering the glaciated portions of the NAIP imagery. In some cases, flights were completed in a single day. For 2006, the inspection date was used, since the start and end dates were not provided.
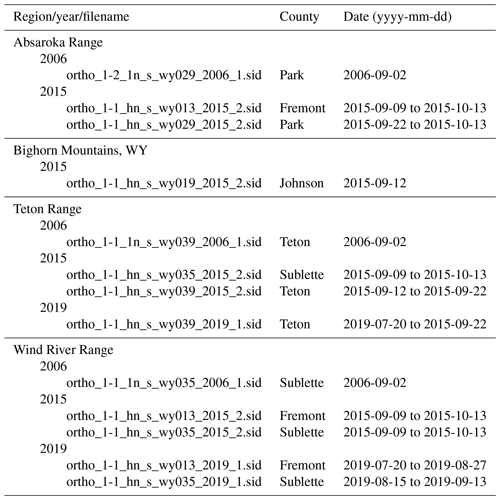
Table A18List of dates of the Maxar imagery used for outlining glaciers and perennial snowfields in Wyoming.
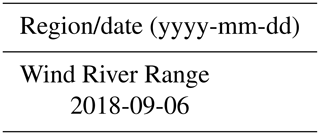
In the southern Wind River Range, a new snow dusting was often present, occasionally making it difficult to outline snowfields and a few glaciers. Distinguishing seasonal snow from perennial snow was a judgment call. If the snow was slightly discolored, similar to underlying rock/soil or looking like the color was coming from underneath, it was identified as seasonal snow. Also, if many snow-free patches (a few square meters) pockmarked the snow or if many rocks protruded through the snow, it was considered seasonal. A perennial patch of snow appeared smooth and white, hiding the underlying surface. Thin snow cover on glacier ice appeared greyish in color and appeared smoother than the surrounding ice-free landscape.
At Lower Fremont Glacier, a number of sizable ice patches appear down the valley as if a deposit of buried ice were present. However, there is no obvious connection to the glacier itself.
The GNIS identified a single glacier as the Sacagawea Glacier and two separate Fremont Glaciers (Fig. A5). By 2017 the single glacier had split into four glaciers. We chose to label the largest glacier and the glacier to the southeast as the Sacagawea Glacier. The other two glaciers were labeled the Fremont Glaciers.
AGF was the principal investigator of the project; he wrote the proposal, digitized glacier and snowfield outlines, analyzed the data, and led the writing of this report. BG was the GIS expert responsible for the geographic format (e.g., projection, attributes, database structure) and quality control. He digitized glacier and snowfield outlines, analyzed the data, and helped write the report. CM provided some of the imagery.
The contact author has declared that none of the authors has any competing interests.
Publisher's note: Copernicus Publications remains neutral with regard to jurisdictional claims in published maps and institutional affiliations.
Hassan Basagic and Kristina Dick digitized the glaciers of Mt. Shasta and the North Cascades National Park, respectively. Taylor Kenton of the University of Waterloo and Scott Beason of Mount Rainier National Park helped us digitize the glaciers of Mount Rainier. We greatly appreciate their help and expertise. Nathan Walker of the US Forest Service provided a very helpful initial review of this report. Any use of trade, firm or product names is for descriptive purposes only and does not imply endorsement by the US Government.
This research has been supported by the US Forest Service (grant no. 20-CS-11132400-229).
This paper was edited by Achim A. Beylich and reviewed by Frank Paul, Tobias Bolch, and Irina Rogozhina.
Alley, R. B., Cuffey, K. M., and Zoet, L. K.: Glacial erosion: status and outlook, Ann. Glaciol., 60, 1–13, https://doi.org/10.1017/aog.2019.38, 2019.
Andreassen, L. M., Nagy, T., Kjøllmoen, B., and Leigh, J. R.: An inventory of Norway's glaciers and ice-marginal lakes from 2018–19 Sentinel-2 data, J. Glaciol., 68, 1085–1106, https://doi.org/10.1017/jog.2022.20, 2022.
Armstrong, R. L.: Mass balance history of Blue Glacier, Washington, USA, in: Glacier Fluctuations and Climatic Change, edited by: Oerlemans, J., Springer Netherlands, 183–192, https://doi.org/10.1007/978-94-015-7823-3_12, 1989.
Bard, J. A.: High-resolution digital elevation dataset for Glacier Peak and vicinity, Washington, based on lidar surveys of August–November, 2014 and June, 2015, https://doi.org/10.5066/F7H41PJG, 2017a.
Bard, J. A.: High-resolution digital elevation dataset for Mt Baker and vicinity, Washington, based on lidar surveys of 2015, https://doi.org/10.5066/F7WD3XR0, 2017b.
Bard, J. A.: High-resolution digital elevation dataset for Mount Adams and vicinity, Washington, based on lidar surveys of August–September, 2016, https://doi.org/10.5066/P9Z1HF1K, 2019.
Beason, S. R., Legg, N. T., Kenyon, T. R., Jost, R. P., and Kennard, P. M.: Forecasting and seismic detection of debris flows in pro-glacial rivers at Mount Rainier National Park, Washington, USA, Contain. Proc. Seventh Int. Conf. Debris-Flow Hazards Mitig. Gold. Colo., USA, 10–13 June 2019, https://doi.org/10.25676/11124/173232, 2018.
Benn, D. I. and Evans, D. J. A.: Glaciers and glaciation, 2nd edn., Hodder Education, London, 802 pp., https://doi.org/10.4324/9780203785010, 2010.
Bolch, T., Menounos, B., and Wheate, R.: Landsat-based inventory of glaciers in western Canada, 1985–2005, Remote Sens. Environ., 114, 127–137, https://doi.org/10.1016/j.rse.2009.08.015, 2010.
Bolch, T., Rohrbach, N., Kutuzov, S., Robson, B. A., and Osmonov, A.: Occurrence, evolution and ice content of ice-debris complexes in the Ak-Shiirak, Central Tien Shan revealed by geophysical and remotely-sensed investigations: Ice-debris complexes in Ak-Shiirak, Earth Surf. Proc. Land., 44, 129–143, https://doi.org/10.1002/esp.4487, 2019.
Bowerman, N. D. and Clark, D. H.: Holocene glaciation of the central Sierra Nevada, California, Quaternary Sci. Rev., 30, 1067–1085, https://doi.org/10.1016/j.quascirev.2010.10.014, 2011.
Brardinoni, F., Scotti, R., Sailer, R., and Mair, V.: Evaluating sources of uncertainty and variability in rock glacier inventories, Earth Surf. Proc. Land., 44, 2450–2466, https://doi.org/10.1002/esp.4674, 2019.
Cadbury, S. L., Hannah, D. M., Milner, A. M., Pearson, C. P., and Brown, L. E.: Stream temperature dynamics within a New Zealand glacierized river basin, River Res. Appl., 24, 68–89, https://doi.org/10.1002/rra.1048, 2008.
Chiarle, M., Iannotti, S., Mortara, G., and Deline, P.: Recent debris flow occurrences associated with glaciers in the Alps, Global Planet. Change, 56, 123–136, https://doi.org/10.1016/j.gloplacha.2006.07.003, 2007.
Cogley, J. G., Hock, R., Rasmussen, L. A., Arendt, A. A., Bauder, A., Braithwaite, R. J., Jansson, P., Kaser, G., Möller, M., Nicholson, L. I., and Zemp, M.: Glossary of Glacier Mass Balance and Related Terms, UNESCO-IHP, Paris, 2011.
Davis, P. T.: Holocene glacier fluctuations in the American Cordillera, Quaternary Sci. Rev., 7, 129–157, 1988.
Denton, G. H.: Conterminous US, Chap. 1, in: Mountain glaciers of the Northern Hemisphere, vol. 1, edited by: Field, W. O., Corps of Engineers, US Army, Technical Information Analysis Center, Cold Regions Research and Engineering Laboratory, 1975.
DeVisser, M. H. and Fountain, A. G.: A century of glacier change in the Wind River Range, WY, Geomorphology, 232, 103–116, https://doi.org/10.1016/j.geomorph.2014.10.017, 2015.
Dewitz, J.: National Land Cover Database (NLCD) 2016 Products (ver. 2.0, July 2020), https://doi.org/10.5066/P96HHBIE, 2019.
Dick, K.: Glacier Change of the North Cascades, Washington 1900–2009, MS thesis, Portland State University, Portland, OR, 127 pp., https://doi.org/10.15760/etd.1062, 2013.
Dussaillant, I., Berthier, E., Brun, F., Masiokas, M., Hugonnet, R., Favier, V., Rabatel, A., Pitte, P., and Ruiz, L.: Two decades of glacier mass loss along the Andes, Nat. Geosci., 12, 802–808, https://doi.org/10.1038/s41561-019-0432-5, 2019.
Earl, L. and Gardner, A.: A satellite-derived glacier inventory for North Asia, Ann. Glaciol., 57, 50–60, https://doi.org/10.3189/2016AoG71A008, 2016.
Evans, I. S.: Local aspect asymmetry of mountain glaciation: A global survey of consistency of favoured directions for glacier numbers and altitudes, Geomorphology, 73, 166–184, https://doi.org/10.1016/j.geomorph.2005.07.009, 2006.
Fagre, D. B., McKeon, L. A., Dick, K. A., and Fountain, A. G.: Glacier margin time series (1966, 1998, 2005, 2015) of the named glaciers of Glacier National Park, MT, USA, https://doi.org/10.5066/f7p26wb1, 2017.
Fellman, J. B., Nagorski, S., Pyare, S., Vermilyea, A. W., Scott, D., and Hood, E.: Stream temperature response to variable glacier coverage in coastal watersheds of Southeast Alaska, Hydrol. Process., 28, 2062–2073, https://doi.org/10.1002/hyp.9742, 2014.
Fischer, A., Seiser, B., Stocker Waldhuber, M., Mitterer, C., and Abermann, J.: Tracing glacier changes in Austria from the Little Ice Age to the present using a lidar-based high-resolution glacier inventory in Austria, The Cryosphere, 9, 753–766, https://doi.org/10.5194/tc-9-753-2015, 2015.
Fountain, A. G. and Glenn, B.: Data From: Inventory of Glaciers and Perennial Snowfields of the Coterminous USA (2022), Portland State University, Portland, OR, https://doi.org/10.15760/geology-data.03, 2022.
Fountain, A. G. and Tangborn, W. V.: The effect of glaciers on streamflow variations, Water Resour. Res., 21, 579–586, 1985.
Fountain, A. G., Hoffman, M. J., Jackson, K., Basagic, H. J., Nylen, T. H., and Percy, D.: Digital outlines and the topography of the Glaciers of the American West, US Geological Survey, https://doi.org/10.3133/ofr20061340, 2007.
Fountain, A. G., Glenn, B., and Basagic, H. J.: The Geography of Glaciers and Perennial Snowfields in the American West, Arct. Antarct. Alp. Res., 49, 391–410, https://doi.org/10.1657/AAAR0017-003, 2017.
Garwood, J. M., Fountain, A. G., Lindke, K. T., van Hattem, M. G., and Basagic, H. J.: 20th Century Retreat and Recent Drought Accelerated Extinction of Mountain Glaciers and Perennial Snowfields in the Trinity Alps, California, Northwest Sci., 94, 44, https://doi.org/10.3955/046.094.0104, 2020.
Gesch, D., Oimoen, M., Greenlee, S., Nelson, C., Steuck, M., and Tyler, D.: The national elevation dataset, Photogramm. Eng. Rem. S., 68, 5–32, 2002.
Heard, J.: Late Pleistocene and Holocene Aged Glacial and Climatic Reconstructions in the Goat Rocks Wilderness, Washington, United States, https://doi.org/10.15760/etd.557, 2000.
Hock, R., de Woul, M., Radić, V., and Dyurgerov, M.: Mountain glaciers and ice caps around Antarctica make a large sea-level rise contribution: Mountain Glaciers and Ice Caps, Geophys. Res. Lett., 36, L07501, https://doi.org/10.1029/2008GL037020, 2009.
Hoffman, M. J., Fountain, A. G., and Achuff, J. M.: 20th-century variations in area of cirque glaciers and glacierets, Rocky Mountain National Park, Rocky Mountains, Colorado, USA, Ann. Glaciol., 46, 349–354, https://doi.org/10.3189/172756407782871233, 2007.
Huss, M. and Hock, R.: Global-scale hydrological response to future glacier mass loss, Nat. Clim. Change, 8, 135–140, https://doi.org/10.1038/s41558-017-0049-x, 2018.
Jin, S., Homer, C., Yang, L., Danielson, P., Dewitz, J., Li, C., Zhu, Z., Xian, G., and Howard, D.: Overall Methodology Design for the United States National Land Cover Database 2016 Products, Remote Sens.-Basel, 11, 2971, https://doi.org/10.3390/rs11242971, 2019.
King, C.: On the discovery of actual glaciers on the Mountains of the Pacific Slope, Am. J. Sci. Arts, 1, 157–167, 1871.
Krimmel, R. M.: Glaciers of the conterminous United States, in: Satellite image atlas of glaciers of the world: North America, edited by: Williams, Jr., R. S. and Ferrigno, J., US Geological Survey Professional Paper, Washington, DC, J329–J381, https://doi.org/10.3133/pp1386j, 2002.
Leigh, J. R., Stokes, C. R., Carr, R. J., Evans, I. S., Andreassen, L. M., and Evans, D. J. A.: Identifying and mapping very small (< 0.5 km2) mountain glaciers on coarse to high-resolution imagery, J. Glaciol., 65, 873–888, https://doi.org/10.1017/jog.2019.50, 2019.
Linsbauer, A., Paul, F., and Haeberli, W.: Modeling glacier thickness distribution and bed topography over entire mountain ranges with GlabTop: Application of a fast and robust approach, J. Geophys. Res., 117, F03007, https://doi.org/10.1029/2011JF002313, 2012.
Linsbauer, A., Huss, M., Hodel, E., Bauder, A., Fischer, M., Weidmann, Y., Bärtschi, H., and Schmassmann, E.: The New Swiss Glacier Inventory SGI2016: From a Topographical to a Glaciological Dataset, Front. Earth Sci., 9, https://doi.org/10.3389/feart.2021.704189, 2021.
Lu, Y., Zhang, Z., Kong, Y., and Hu, K.: Integration of optical, SAR and DEM data for automated detection of debris-covered glaciers over the western Nyainqentanglha using a random forest classifier, Cold Reg. Sci. Technol., 193, 103421, https://doi.org/10.1016/j.coldregions.2021.103421, 2022.
Meier, M. F.: Distribution and variations of glaciers in the United States exclusive of Alaska, Int. Assoc. Sci. Hydrol., 54, 420–429, 1961.
Meier, M. F.: Contribution of small glaciers to global sea level, Science, 226, 1418–1421, 1984.
Mishra, A., Nainwal, H. C., Bolch, T., Shah, S. S., and Shankar, R.: Glacier inventory and glacier changes (1994–2020) in the Upper Alaknanda Basin, Central Himalaya, J. Glaciol., 69, 591–606, https://doi.org/10.1017/jog.2022.87, 2023.
Moore, R. D., Fleming, S. W., Menounos, B., Wheate, R., Fountain, A., Stahl, K., Holm, K., and Jakob, M.: Glacier change in western North America: influences on hydrology, geomorphic hazards and water quality, Hydrol. Process., 23, 42–61, https://doi.org/10.1002/hyp.7162, 2009.
NAIP: National agricultural imagery program (NAIP) Information Sheet, United States Department of Agriculture, 2017.
O'Connor, J. E., Hardison, J. H., and Costa, J. E.: Debris flows from failures of Neoglacial-age moraine dams in the Three Sisters and Mount Jefferson wilderness areas, Oregon: a study of the recent debris flows from moraine-dammed lake releases at central Oregon Cascade Range volcanoes – Mt. Jefferson, Three Fingered Jack and the Three Sisters/Broken Top, US Geological Survey, Reston, VA, 93 pp., https://doi.org/10.3133/pp1606, 2001.
Osborn, G., Menounos, B., Ryane, C., Riedel, J. L., Clague, J. J., Koch, J., Clark, D., Scott, K., and Davis, P. T.: Latest Pleistocene and Holocene glacier fluctuations on Mount Baker, Washington, Quaternary Sci. Rev., 49, 33–51, https://doi.org/10.1016/j.quascirev.2012.06.004, 2012.
Parkes, D. and Marzeion, B.: Twentieth-century contribution to sea-level rise from uncharted glaciers, Nature, 563, 551–554, https://doi.org/10.1038/s41586-018-0687-9, 2018.
Paul, F., Kääb, A., and Haeberli, W.: Recent glacier changes in the Alps observed by satellite: Consequences for future monitoring strategies, Global Planet. Change, 56, 111–122, https://doi.org/10.1016/j.gloplacha.2006.07.007, 2007.
Paul, F., Barrand, N. E., Baumann, S., Berthier, E., Bolch, T., Casey, K., Frey, H., Joshi, S. P., Konovalov, V., Le Bris, R., Mölg, N., Nosenko, G., Nuth, C., Pope, A., Racoviteanu, A., Rastner, P., Raup, B., Scharrer, K., Steffen, S., and Winsvold, S.: On the accuracy of glacier outlines derived from remote-sensing data, Ann. Glaciol., 54, 171–182, https://doi.org/10.3189/2013AoG63A296, 2013.
Paul, F., Rastner, P., Azzoni, R. S., Diolaiuti, G., Fugazza, D., Le Bris, R., Nemec, J., Rabatel, A., Ramusovic, M., Schwaizer, G., and Smiraglia, C.: Glacier shrinkage in the Alps continues unabated as revealed by a new glacier inventory from Sentinel-2, Earth Syst. Sci. Data, 12, 1805–1821, https://doi.org/10.5194/essd-12-1805-2020, 2020.
Pfeffer, W. T., Arendt, A. A., Bliss, A., Bolch, T., Cogley, J. G., Gardner, A. S., Hagen, J.-O., Hock, R., Kaser, G., and Kienholz, C.: The Randolph Glacier Inventory: a globally complete inventory of glaciers, J. Glaciol., 60, 537–552, https://doi.org/10.3189/2014JoG13J176, 2014.
Post, A., Richardson, D., Tangborn, W. V., and Rosselot, F.: Inventory of glaciers in the North Cascades, Washington, USGS Professional Paper 705-A, US Geological Survey, Washington, DC, 1971.
Pritchard, H. D.: Asia's shrinking glaciers protect large populations from drought stress, Nature, 569, 649–654, https://doi.org/10.1038/s41586-019-1240-1, 2019.
Rabatel, A., Sirguey, P., Drolon, V., Maisongrande, P., Arnaud, Y., Berthier, E., Davaze, L., Dedieu, J.-P., and Dumont, M.: Annual and Seasonal Glacier-Wide Surface Mass Balance Quantified from Changes in Glacier Surface State: A Review on Existing Methods Using Optical Satellite Imagery, Remote Sens.-Basel, 9, 507, https://doi.org/10.3390/rs9050507, 2017.
Rasmussen, L. A.: South Cascade Glacier mass balance, 1935–2006, Ann. Glaciol., 50, 215–220, 2009.
RGIK: Towards standard guidelines for inventorying rock glaciers: baseline concepts (version 4.2.2), IPA Action Group Rock glacier inventories and kinematics, https://doi.org/10.5194/icg2022-478, 2022.
Robinson, J. E.: Digital topographic data based on lidar survey of Mount Shasta Volcano, California, July–September 2010, Reston, VA, https://doi.org/10.3133/ds852, 2014.
Robson, B. A., Bolch, T., MacDonell, S., Hölbling, D., Rastner, P., and Schaffer, N.: Automated detection of rock glaciers using deep learning and object-based image analysis, Remote Sens. Environ., 250, 112033, https://doi.org/10.1016/j.rse.2020.112033, 2020.
Russell, I. C.: The glaciers of North America, Geogr. J., 12, 553–564, 1898.
Schiefer, E., Menounos, B., and Wheate, R.: Recent volume loss of British Columbian glaciers, Canada: Volume loss of BC glaciers, Geophys. Res. Lett., 34, L16503, https://doi.org/10.1029/2007GL030780, 2007.
Selkowitz, D. J. and Forster, R. R.: Automated mapping of persistent ice and snow cover across the western U. S. with Landsat, ISPRS J. Photogramm., 117, 126–140, https://doi.org/10.1016/j.isprsjprs.2016.04.001, 2016.
Sitts, D. J., Fountain, A. G., and Hoffman, M. J.: Twentieth Century Glacier Change on Mount Adams, Washington, USA, Northwest Sci., 84, 378–385, https://doi.org/10.3955/046.084.0407, 2010.
Smiraglia, C., Azzoni, R. S., D'Agata, C., Maragno, D., Fugazza, D., and Diolaiuti, G. A.: The New Italian Glacier Inventory: a didactic tool for a better knowledge of the natural Alpine environment, J-Read.-J. Res. Didact. Geogr., 4, https://doi.org/10.4458/5196-08, 2015.
Spicer, R.: Glaciers in the Olympic Mountains, Washington – Present distribution and recent variations, MS thesis, University of Washington, Seattle, WA, 158 pp., 1986.
Sun, M., Liu, S., Yao, X., Guo, W., and Xu, J.: Glacier changes in the Qilian Mountains in the past half-century: Based on the revised First and Second Chinese Glacier Inventory, J. Geogr. Sci., 28, 206–220, https://doi.org/10.1007/s11442-018-1468-y, 2018.
Trcka, A.: Inventory of Rock Glaciers in the American West and Their Topography and Climate, https://doi.org/10.15760/etd.7509, 2020.
Usery, E. L., Varanka, D., and Finn, M. P.: A 125 year history of topographic mapping and GIS in the U. S. Geological Survey 1884–2009, part 1: 1884–1980, ArcNews, 31, p. 39, 2009.
Wickham, J., Stehman, S. V., Sorenson, D. G., Gass, L., and Dewitz, J. A.: Thematic accuracy assessment of the NLCD 2016 land cover for the conterminous United States, Remote Sens. Environ., 257, 112357, https://doi.org/10.1016/j.rse.2021.112357, 2021.
Yao, T., Pu, J., Lu, A., Wang, Y., and Yu, W.: Recent glacial retreat and its impact on hydrological processes on the Tibetan Plateau, China, and surrounding regions, Arct. Antarct. Alp. Res., 39, 642–650, 2007.
Zalazar, L., Ferri, L., Castro, M., Gargantini, H., Gimenez, M., Pitte, P., Ruiz, L., Masiokas, M., Costa, G., and Villalba, R.: Spatial distribution and characteristics of Andean ice masses in Argentina: results from the first National Glacier Inventory, J. Glaciol., 66, 938–949, https://doi.org/10.1017/jog.2020.55, 2020.
Zemp, M., Huss, M., Thibert, E., Eckert, N., McNabb, R., Huber, J., Barandun, M., Machguth, H., Nussbaumer, S. U., Gärtner-Roer, I., Thomson, L., Paul, F., Maussion, F., Kutuzov, S., and Cogley, J. G.: Global glacier mass changes and their contributions to sea-level rise from 1961 to 2016, Nature, 568, 382–386, https://doi.org/10.1038/s41586-019-1071-0, 2019.