the Creative Commons Attribution 4.0 License.
the Creative Commons Attribution 4.0 License.
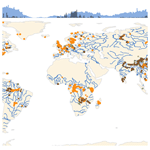
GRiMeDB: the Global River Methane Database of concentrations and fluxes
Luke C. Loken
Nora J. Casson
Samantha K. Oliver
Ryan A. Sponseller
Marcus B. Wallin
Liwei Zhang
Gerard Rocher-Ros
Despite their small spatial extent, fluvial ecosystems play a significant role in processing and transporting carbon in aquatic networks, which results in substantial emission of methane (CH4) into the atmosphere. For this reason, considerable effort has been put into identifying patterns and drivers of CH4 concentrations in streams and rivers and estimating fluxes to the atmosphere across broad spatial scales. However, progress toward these ends has been slow because of pronounced spatial and temporal variability of lotic CH4 concentrations and fluxes and by limited data availability across diverse habitats and physicochemical conditions. To address these challenges, we present a comprehensive database of CH4 concentrations and fluxes for fluvial ecosystems along with broadly relevant and concurrent physical and chemical data. The Global River Methane Database (GriMeDB; https://doi.org/10.6073/pasta/f48cdb77282598052349e969920356ef, Stanley et al., 2023) includes 24 024 records of CH4 concentration and 8205 flux measurements from 5029 unique sites derived from publications, reports, data repositories, unpublished data sets, and other outlets that became available between 1973 and 2021. Flux observations are reported as diffusive, ebullitive, and total CH4 fluxes, and GriMeDB also includes 17 655 and 8409 concurrent measurements of concentrations and 4444 and 1521 fluxes for carbon dioxide (CO2) and nitrous oxide (N2O), respectively. Most observations are date-specific (i.e., not site averages), and many are supported by data for 1 or more of 12 physicochemical variables and 6 site variables. Site variables include codes to characterize marginal channel types (e.g., springs, ditches) and/or the presence of human disturbance (e.g., point source inputs, upstream dams). Overall, observations in GRiMeDB encompass the broad range of the climatic, biological, and physical conditions that occur among world river basins, although some geographic gaps remain (arid regions, tropical regions, high-latitude and high-altitude systems). The global median CH4 concentration (0.20 µmol L−1) and diffusive flux (0.44 ) in GRiMeDB are lower than estimates from prior site-averaged compilations, although ranges (0 to 456 µmol L−1 and −136 to 4057 ) and standard deviations (10.69 and 86.4) are greater for this larger and more temporally resolved database. Available flux data are dominated by diffusive measurements despite the recognized importance of ebullitive and plant-mediated CH4 fluxes. Nonetheless, GriMeDB provides a comprehensive and cohesive resource for examining relationships between CH4 and environmental drivers, estimating the contribution of fluvial ecosystems to CH4 emissions, and contextualizing site-based investigations.
- Article
(5776 KB) - Full-text XML
-
Supplement
(605 KB) - BibTeX
- EndNote
Despite their small areal extent, running-water (fluvial) ecosystems play a significant role in processing and transporting carbon (C) in and through aquatic networks, including the production, consumption, transport, and evasion of carbon dioxide (CO2) and methane (CH4). The profound planetary warming effects of CH4 in the atmosphere, its erratic but accelerating rate of increase over recent years (NOAA, 2022), the significant contributions of natural sources to the growing atmospheric pool (Turner et al., 2019), and improvements in gas measurement technologies have all contributed to a rapid increase in studies of CH4 dynamics in aquatic environments in general and fluvial ecosystems in particular. These studies reveal widespread supersaturation of CH4 in running waters that underlies their larger-than-expected contribution to the atmospheric pool (Stanley et al., 2016).
Efforts to quantify fluvial CH4 dynamics at regional, continental, and global scales have been fraught with uncertainty, reflecting the inherent variability of this gas in surface waters combined with a notable limitation in data availability. Sources and sinks of CH4 are often unevenly distributed over space and time within drainage systems and, as a result, concentrations can vary over 1–3 orders of magnitude over short time periods (subdaily to subweekly; e.g., Natchimuthu et al., 2017; Smith and Böhlke, 2019) or relatively small spatial extents (< 10 to < 100 m for small streams and large rivers; e.g., Anthony et al., 2012; Crawford et al., 2017; Bretz et al., 2021; Robison et al., 2021). Similarly, several drivers or predictors of CH4 have been identified in the literature, and these properties also have variable spatial and temporal distributions. Thus, efforts to estimate the total emissions from world rivers have relied on relatively small data sets composed of site-specific values that have been averaged over time and have then used upscaling strategies based on Monte Carlo techniques or extrapolations using predictor variables that have little or no significant statistical relationships with large-scale patterns of gas concentrations or fluxes (Hutchins et al., 2020). Consequently, current global-scale estimates of riverine emissions are poorly constrained and highly uncertain (Saunois et al., 2020; Rosentreter et al., 2021).
The combination of rapidly increasing atmospheric concentrations of CH4, the significant role of fluvial systems in emitting this gas, and, critically, current difficulties in explaining or predicting concentrations and fluxes with reasonable certainty inspired the central goal of this paper: to assemble a comprehensive database of CH4 concentrations and fluxes for fluvial ecosystems that includes broadly relevant concurrent physical and chemical data. This effort expands upon a prior compilation of CH4 and CO2 data (named MethDB; Stanley et al., 2015) that was constructed to emphasize among-site differences and included 1496 concentration records and 532 flux records from 1080 sites. In this more comprehensive Global River Methane Database (GRiMeDB), most data are date-specific (i.e., not averaged over time), the breadth of site types is expanded to include marginal fluvial habitats as well as disturbed and artificial waterways, and CH4 data are supported by a broad suite of site-specific physical and chemical attributes along with concurrent measurements of CO2 and N2O where available. Given the more finely resolved scale of the data and the growth of the field in the past decade, GRiMeDB represents a significant expansion beyond MethDB. Building GRiMeDB with greater detail and breadth of data was done with the intent of increasing opportunities to identify and predict spatial and temporal variation in CH4, to test hypotheses related to greenhouse gas dynamics, and to reduce uncertainty in future upscaled estimates of gas emissions. In this paper, we (1) provide a detailed description of the components of the database and its construction, (2) summarize some basic patterns of gas concentrations and fluxes from GRiMeDB, and (3) highlight critical data gaps and possible future research opportunities for improving current understanding of CH4 dynamics in streams and rivers.
GRiMeDB is composed of four tables that contain information related to (1) data sources, (2) sites, (3) gas concentrations and supporting physicochemical data, and (4) gas fluxes. All tables are linked by unique data source identifiers, and all concentration and flux observations are also linked to unique site numbers (Fig. 1). Data included in GRiMeDB were gathered from scientific journals, government reports, public data repositories, theses, dissertations, and unpublished data sets provided by individual investigators. Sources were discovered via searches of bibliographic databases and data repositories (Web of Science, Google Scholar, ProQuest Dissertations and Theses Global, China National Knowledge Infrastructure, Environmental Data Initiative, USGS ScienceBase, Natural Environment Research Council (NERC) Environmental Information Data Centre, Arctic Data Center, PANGAEA, Zenodo) using the keywords methane and stream* or river* or ditch* or canal*, and searches were repeated numerous times prior to December 2021 for completeness. We also used informal “word-of-mouth” approaches to discover additional, often unpublished data sets.
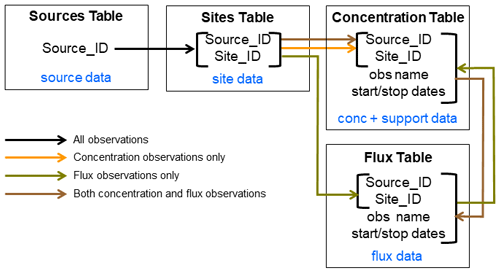
Figure 1General structure of GRiMeDB and connections between its four tables. Information flow began with entering information about each data source into the source table and assigning a unique Source_ID. Site information for each site within a data source was then entered into the site table. The site was given a unique Site_ID and linked to its data source by the Source_ID. Source_IDs and Site_IDs were carried over to all concentration and flux observations in their respective tables. Methane (CH4) observations include site–date combinations with only concentration data (orange), only flux data (green), or both concentration and flux data (brown). Concentrations and available supporting data (described in Sect. 2.3) were entered into the concentration table, and each observation was given a unique observation (obs) name. For site–date combinations that had both concentration and flux observations, the Source_ID, Site_ID, observation name, and date information were copied to the flux table for data entry. Site–date combinations with flux data only were entered into the flux table and given a unique observation name. If a flux observation had associated supporting data, the Source_ID, Site_ID, observation name, and date information were copied to the concentration table for supporting data entry. However, if there were no supporting data, matching rows were not added to the concentration table.
All potential data sources were first screened to determine their appropriateness for inclusion in GRiMeDB. Several criteria were established a priori to ensure the usability of the data and that they were derived from inland running water systems. Coastal sites with > 1 ppt salinity were considered estuarine and thus were excluded. Similarly, sites that were situated in reservoirs or immediately upstream of small dams, dam spillways, beaver ponds, or lake outlets or that were subject to experimental manipulation were omitted. We did not enter fluxes derived from chambers attached to collars or inserted into sediments because we could not be certain that such measurements were capturing air–water fluxes. Sources that reported minimum and maximum gas concentrations or fluxes only were not included. Finally, rates expressed on an annual basis were also excluded to avoid introducing uncertainty associated with different upscaling assumptions and methods.
2.1 Source table
The source table contains the list of all sources used to build GRiMeDB, a unique identification number (Source_ID) for each CH4 data source, and basic bibliographic information for the data source (Title, Author, Source, publication year Pub_year, and digital object identifiers Paper_DOI or Data_DOI_primary or another persistent identifier; all column titles for this table are defined in Table A1). In several cases, data sources were supplemented with additional supporting information (e.g., associated physicochemical data) from separate sources (described further in Sect. 2.3) or additional or corrected information from authors (Fig. 2). In the latter case, we contacted authors when questions arose regarding their data (e.g., clarification regarding units) and/or to request supporting information or site- or date-specific concentrations or fluxes if published values were aggregated. Inclusion of additional unpublished data from authors is noted in the source table along with a description of the addition or correction. If supporting data from separate published sources were used, the DOI or another persistent identifier for the secondary source was listed in a separate column (Data_DOI_supporting).
2.2 Site table
The site table reports basic information on attributes for all sites where CH4 was sampled. Each site has a unique identification code (Site_ID) and name (usually taken directly from the data source) and is linked to the source table via the Source_ID (see Table A2 for detailed descriptions of all columns in the site table). What comprises a “site” (i.e., the spatial extent of data collection) varied among data sources and includes (1) discrete sampling points, (2) geomorphically distinct study reaches, and/or (3) larger channel sections, drainage networks, or other geographic units. The second case typically corresponded to reaches such as riffles or pools in small streams. In the third case, multiple points were often sampled within the “site” and data were then presented as averages. The distance between sampling points that had been averaged varied widely but were typically > 1 km and in some cases exceeded 100 km. Because land use, channel order, and slope can vary substantially across such distances, we included fields to indicate whether a site was an aggregation of widespread points (“aggregated”) and, if so, the number of locations in the aggregation (if available). We also limited the resolution of latitude and longitude for these sites to less than three decimal places. At the opposite extreme, gas sampling at points very close to one another (a “high-density site” sensu Fig. 3) has the potential to create ambiguities for site delineation and data analysis. To avoid these pitfalls, we combined points with slightly different latitude–longitude values to represent a single site for three specific cases. First, multiple samples collected at different points and/or depths within a channel cross section were averaged to form a single site. Second, some drainages or regions were surveyed repeatedly (particularly the Congo River basin and streams in Pennsylvania, USA), and it was not always clear whether closely situated (ca. 10–50 m) points from different surveys were intended to be a repeated sampling of the same location or sampling of discrete sites. Some judgment was involved in choosing between these two possibilities, and in a subset of cases, points in close proximity to one another that were sampled on separate dates were treated as a single site. What constituted “close proximity” varied between small streams and large rivers but was always < 100 m and typically < 50 m. Finally, three data sources had extremely high sampling densities within discrete reaches (50 to > 20 000 samples per reach; Crawford et al., 2016; Call et al., 2018; Loken et al., 2018). Because closely adjacent gas samples can be spatially autocorrelated (Crawford et al., 2017) and including all individual values from these studies would have resulted in their overrepresentation in the database, individual point measurements were treated as within-reach replicates.
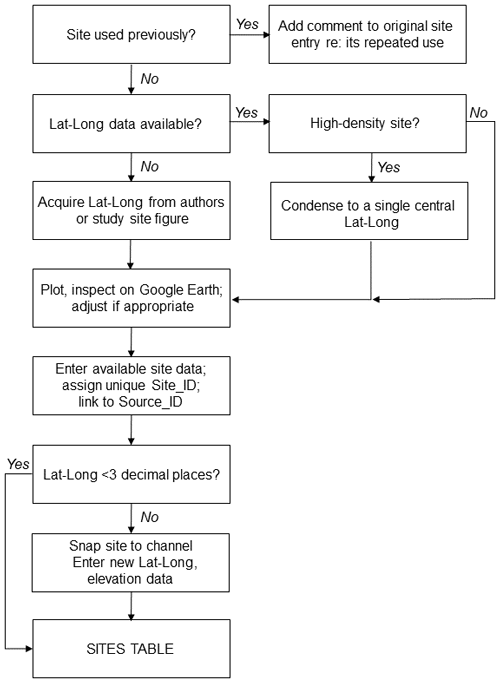
Figure 3Workflow for entering and checking data for the GRiMeDB site table. “Lat–long” is an abbreviation for latitude and longitude.
For a site used in multiple studies, the Site_ID was assigned to the earliest paper and a comment was added to the site entry noting its use in other data sources (Fig. 3). Latitude and longitude coordinates were available for most sites; however, in several cases, location information was acquired from authors or estimated from study site figures using Google Earth (© Google Earth, 2020, https://earth.google.com/, last access: 10 April 2023). All sites were plotted on Google Earth and inspected (Fig. 3) to identify and correct data errors. If a site's coordinates were immediately adjacent to but not on a channel, the coordinates were adjusted to fall on the channel, and this modification was noted in the Comments field. If available, additional variables drawn from the data sources were entered to characterize the site, including stream name, basin or region name, elevation, channel slope, Strahler order, basin area, and codes denoting distinct channel or site types (described below). To supplement the available elevation data, we also estimated elevation for all sites except aggregated sites or sites with poorly resolved coordinates (fewer than three decimal places for both latitude and longitude) after snapping coordinates to the nearest stream. To determine the adjusted within-channel coordinates, we first downloaded a digital elevation model (DEM) for each site using the function get_elev_raster() from the package “elevatr” (version 0.4.2; Hollister et al., 2021) for R statistical software (version 4.2, R Core Team 2021) at a resolution of 6–9 m depending on the location on the globe. Second, the DEM was processed for hydrological correctness using the package “whitebox” (version 1.2.0, Wu, 2020) by filling single-cell pits (fill_single_cell_pits() function) and breaching depressions (breach_depressions() function) to obtain a flow-direction model (d8_pointer() function). Finally, we calculated a flow-accumulation model (d8_flow accumulation() function). If the coordinates reported in the data source had a flow accumulation of < 10 cells (indicating that they were not located in a preferential flow path), new coordinates were assigned to the cell with the highest flow accumulation within a 50 m radius. If the initial site had a high flow-accumulation value (> 10 cells), we assumed that the site was in a stream channel. Typically, the snapping procedure resulted in very minor changes to a site's location (median < 3 m).
Table 1Codes denoting distinct site or channel attributes or the presence of conditions that potentially affect methane (CH4) concentrations or fluxes. Assignment of codes to a site is based on information provided in the data source and/or physical appearance of a site, and a site may have more than one code. Codes are reported in the Channel_type field of the site table.
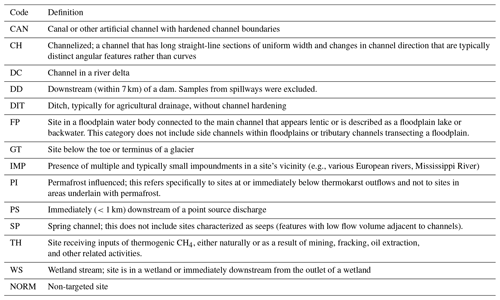
Many studies of CH4 dynamics have been undertaken to determine whether and how specific phenomena such as the presence of upstream reservoirs, point source discharges, thermokarst features, or oil and gas extraction potentially affect fluvial CH4 (and other constituents), usually with an expectation of a net enhancement of concentrations and fluxes. Similarly, other studies have examined sites that may be expected to be enriched in CH4 but whose fluvial identity might be considered marginal or ambiguous (e.g., springs, floodplain backwaters, ditches, canals). Inclusion of such “methane hunting” studies has the potential to bias the data set toward higher values (Stanley et al., 2016). Nonetheless, we included these studies in GRiMeDB because they provide an opportunity to investigate the consequences of human activity and gain a more comprehensive understanding of fluvial CH4 dynamics. However, to accommodate future analyses in which use of such data might be unsuitable, or alternatively, when these sites might be the sole focus of a study, we generated a set of channel codes to identify targeted site types (Table 1). Information about four of the codes was not consistently available among data sources, and thus their assignment often involved judgment calls. The first case involved determining whether the presence of an upstream dam (code DD) was relevant for sites of varying distances downstream. We used a distance of 7 km as a cutoff for this category, although the zone of influence may be far shorter or extend far beyond this distance depending on dam size and operation (Kemenes et al., 2007), respectively. To provide some context for this code, a site's distance from a dam was acquired from the data source or estimated in Google Earth using the Path tool and reported in the Comments field whenever possible. The second case involved straight, symmetrical channels that are common in many agricultural and urban areas. Frequently, it was not known whether this unnatural geometry was due to channelization (straightening) of a stream (code CH) or creation of a new channel (ditches and canals; codes DIT and CAN). In the absence of specific information, straight channels were classified as CH. Third, channels draining or passing through wetlands (WS) were often difficult to identify, particularly given seasonal variation in wetland appearance. Finally, floodplain channels presented a distinct challenge because of the complex nature of these environments and their potential to be classified as either riverine or wetland systems. We used the floodplain (FP) code to indicate habitats that were described as or appeared to be lentic (i.e., backwaters or connected floodplain lakes) but that were persistently connected to the main river channel and thus were part of the fluvial system. Given these ambiguities, we recommend that these four codes be viewed and used with care.
2.3 Concentration table and flux table
The concentration table and the flux table contain the primary gas data central to GRiMeDB, and the concentration table also hosts physical and chemical variables associated with concentration and/or flux observations (see Tables A3 and A4 for the full list of columns and their descriptions). The vast majority of concentration and flux data were extracted from tables within data sources or data repositories or were provided by authors. However, in some cases, values were acquired from figures using graphical digitizing software (WebPlotDigitizer, https://automeris.io/WebPlotDigitizer/, last access: 10 April 2023, GetData, http://getdata-graph-digitizer.com/, last access: 10 April 2023, or DigitizeIt, https://www.digitizeit.xyz/, last access: 10 April 2023). Plots with log scales or that were difficult to interpret were not digitized. The accuracy and consistency of this method were evaluated by comparing data generated by different individuals digitizing a set of common figures and by comparing digitized results to known results. Agreement between both comparisons was strong (average slope = 0.994, average R2 = 0.9996 for five comparisons between individuals digitizing the same data set and average slope = 0.998, average R2 = 0.997 for digitized versus actual data for seven data sets; see Table S1 in the Supplement for further details), demonstrating the reliability of this method of data gathering.
Whenever possible, concentrations and fluxes were entered as values for individual sites on individual days (i.e., not averaged across sites or days) (Fig. 4). Because 1 d represented the lowest level of temporal resolution in GRiMeDB, repeated measurements made on a subdaily scale were averaged and expressed as a daily value and were not considered to be aggregated over time. If multiple replicates were collected at different times on the same day (e.g., a study of diurnal gas dynamics), this was noted in the Comments field, and measurements prior to and after 12:00 local time (LT) were entered as separate, consecutive days. Observations resolved to the daily scale can be identified using either a “No” in the Aggregated_Time field or by having the same reported starting (Date_start) and ending (Date_end) dates. If the specific start and end dates were not specified in the data source, we entered the day as the 15th of the month and noted this approximation in the Comments field. If available, we also reported minimum and maximum values and standard deviations (SDs) for entries that were aggregated over space and/or time. SDs but not minima and maxima were reported for replicates from non-aggregated sampling when available, except for reach-averaged entries with multiple within-reach measurements and diel studies with multiple within-day values. In these cases, minima and maxima were also included.
Dealing with concentration data reported as a negative value, zero, or below a detection limit (BDL) is problematic because of inconsistencies in detection limits and reporting practices, and any decision about handling these records introduces some bias (Stow et al., 2018). For example, using a non-numerical format such as BDL or < 0.01 is likely to lead to the elimination of these entries during data analysis and thus would introduce a bias against low-value observations. Alternatively, converting any such value to zero would introduce a bias in the opposite direction. As a compromise solution, concentrations recorded as zero in the original data source were entered as zero in GRiMeDB, and other below-detection values were entered as −999 999. In this latter case, the original data entry format was noted in the Comments column. For fluxes, negative and zero values were entered without modification or comment.
The flux table reports diffusive, ebullitive, and total CH4 fluxes along with CO2 and N2O diffusive fluxes. Given the diverse strategies for measuring each of the three CH4 flux pathways and potential biases associated with different approaches (Lorke et al., 2015; Chen et al., 2021), values are accompanied by brief categorical descriptions of methods used for each flux type as well as for CO2 fluxes and the gas exchange coefficient k. For a small number of entries, CH4 fluxes were not directly reported in the data source, but information was available (dissolved gas concentration, temperature, and a corresponding k value) that allowed us to calculate these fluxes. We also entered BDL values for flux for one data source in which fluxes had been calculated from concentration, but fluxes associated with BDL concentrations had been omitted from the results. Finally, a small number of observations listed diffusive and ebullitive but not total fluxes, so diffusion and ebullition were summed and entered as total flux. In all cases, the added calculations are noted in the Comments field.
The GRiMeDB concentration table includes physicochemical measurements in support of concentration and flux observations (Figs. 1 and 4, Table A3). Availability of this supplemental information varied widely among data sources and was limited to data collected concurrently with gas samples. For data sources with gas fluxes and physicochemical data but not gas concentrations, we created rows in the concentration table to capture the supporting data. These records are identified by a “Yes” in the FluxYesNo column, SampleCount = 0, and NA in the CH4mean column. Finally, water temperature was estimated for entries if it was needed to convert gas units and entered in the WaterTemp_degC_estimated column. Estimates were typically based on values from adjacent sites or the same site at a similar time (e.g., averages of temperature from the prior and subsequent dates or from the same month in an adjacent year). Error introduced from these estimates should be small, e.g., ca. < 10 % of the actual value if the estimated temperature is off by 3 ∘C.
Following completion of all data entry, gas and physicochemical variables were converted to “new” standard units (Tables A3 and A4). The identities of the new and original units are included in both the concentration table and flux table for clarity. Elevation was used to estimate atmospheric pressure if needed for unit conversions. We used Henry's law, water temperature, and atmospheric pressure to convert reported dissolved gas values (ppm, ppb, µatm, and percentage saturation; ∼ 13 % of observations). For observations that reported gas values as percentage saturation (< 1 % of all observations), we also used the global average CH4, CO2, and the N2O atmospheric concentrations from the NOAA Global Monitoring Laboratory (https://gml.noaa.gov/ccgg/, last access: 12 June 2023) for the year 2013, which corresponds to the median observation year in the database.
2.4 Assessment of representativeness
We assessed the representativeness of sites in GRiMeDB relative to the global distribution of biological, physical, and climatic properties following van den Hoogen et al. (2021). Briefly, we first assigned each site to a corresponding river reach in HydroSHEDS (Linke et al., 2019), which is a global hydrological network database that contains spatial data for a wide array of hydrological, physiographical, climatic, land cover, geological, edaphic, and anthropogenic variables for each river reach. HydroSHEDS thus provides a multidimensional characterization of global rivers that is well suited for assessing how representative GRiMeDB sites are in terms of key biophysical and anthropogenic features. After excluding non-numerical variables (e.g., biome) and variables with monthly values (e.g., monthly precipitation), we performed a principal component analysis (PCA) on all HydroSHEDS subcatchments using all possible combinations of the 54 remaining HydroSHEDS variables. From this, we selected all principal components (PCs) needed to explain 90 % of the variance in the PCA, which corresponded to 28 PCs and 378 possible bivariate combinations of these PCs. For each unique PC pair, we computed the convex hull of all sampled sites to determine the distribution of these sites relative to all global river subcatchments for the specified PCs (Fig. 5). Each HydroSHEDS subcatchment was then assigned a value of 1 or 0 if it fell within or outside the convex hull, respectively. This process was repeated for each of the 378 possible PC combinations. To collapse this information, we calculated the fraction of cases where a given subcatchment fell within the convex hull for all PC combinations to obtain a dimensionless summary value ranging from 0 to 1. A subcatchment with a value of 1 for this index of “representativeness” means that it fell within the convex hull for 100 % of the PC combinations, indicating that its overall characteristics are well captured in the database. It is important to note that this analysis only captures average catchment properties of relatively large river reaches (average subcatchment area: 130 km2). Given the strong local controls on CH4 concentrations and fluxes, interpretations from this analysis should be made with some caution.
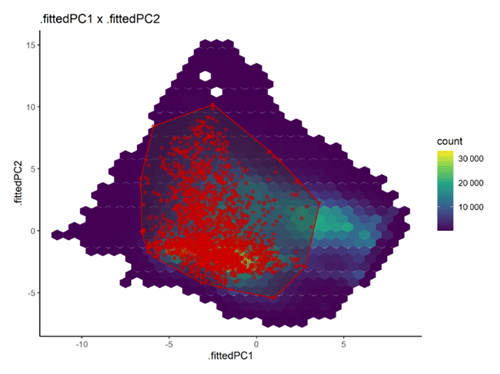
Figure 5Example of a representative principal component analysis (PCA) hexagon plot based on variability in HydroSHEDS river subcatchment attributes. Hexagon color indicates the number of subcatchments per hexagon. Subcatchments hosting GRiMeDB sites are plotted in red and contained within the convex hull delineated by red lines. Subcatchments that fall within this polygon are assigned a value of 1, and those outside the perimeter are given a value of 0 to indicate the representativeness of sampled reaches for this pair of PC axes. See Sect. 2.4 for further explanation.
2.5 Data checking and data analysis
Several approaches were taken to check the accuracy of data in GRiMeDB. This included evaluation of the reliability of digitized data (Sect. 2.3) along with several additional inspection steps. Entries were error checked by a coauthor other than the individual who entered the data, including confirmation of site location information, validation of units for all variables, and spot or complete checking of entered gas data (independent units and data check in Fig. 4), depending on dataset length and whether data were manually entered or imported directly from a file. Once values had been converted to standard units, all variables were plotted to identify outliers (outlier check; Fig. 4), and extreme values were checked against the original data source. In cases in which errors were present in the original data, if possible, authors were contacted for clarification. In the few rare cases in which issues could not be resolved, the data were excluded. These and all other calculations and analyses were performed in R (version 4.2, R Core Team 2021) using the “dplyr” (version 1.0.7, Wickham et al., 2021) and “data.table” (Dowle and Srinivasan, 2021) packages for data analysis, the “sf” package (version, 1.0, Pebesma, 2018) for spatial data processing, and the “ggplot2” (version 3.3.5, Wickham, 2016) and “patchwork” (Pedersen, 2022) packages for visualization.
3.1 Overview of GRiMeDB data
GRiMeDB includes 24 024 records of CH4 concentration and 8205 CH4 flux values from 5037 unique sites along with 17 655 and 8409 concurrent measurements of concentration and 4444 and 1521 of flux for CO2 and N2O, respectively (Table S2). Although the first concentration and flux values in GRiMeDB were published in 1973 (Lamontagne et al., 1973) and 1987 (de Angelis and Lilley, 1987), respectively, over 70 % of all CH4 concentrations and 80 % of flux observations became available after 2015 (the year of publication of MethDB; Fig. 6, Fig. S1 in the Supplement). This growth in data availability has occurred predominantly along the spatial axis, as almost two-thirds of all the sites were added in or after 2015 and over half of all the sites in the database have a single concentration and/or flux observation. Conversely, long time series are rare, with only 8 % of the 5037 sites having > 10 concentration observations and 4 % having > 10 diffusive flux records (Figs. 6 and S1). The longest concentration record includes 590 observations distributed over 28 years (Toolik Inlet, Site_ID 9025; Kling, 2019a; Kling, 2022), while the longest flux record has 82 observations of diffusive flux over 4 years (Site_ID 3644; Aho et al., 2021). Further, among the 15 sites with time series > 5 years, 12 are situated in either the Toolik Lake region of Alaska, USA (Kling, 2019a, b, 2022), or within the Krycklan watershed in Sweden (Wallin et al., 2018).
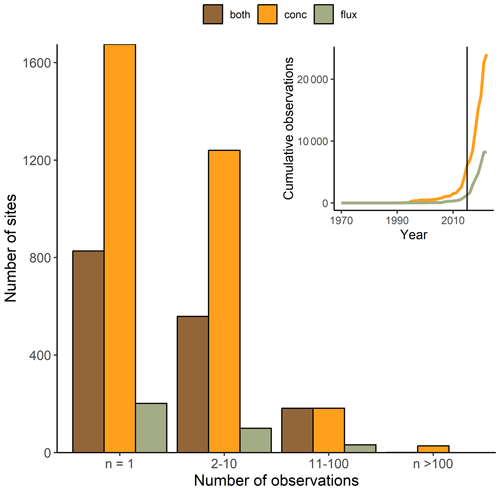
Figure 6Distribution of the number of methane (CH4) observations per site. Brown bars indicate sites with both concentration (conc) and flux observations. Orange and green bars show sites with only concentration and only flux observations, respectively. Inset: cumulative observations of CH4 concentration and flux data based on the year of publication of the data source. The vertical line (2015) indicates the year of MethDB (Stanley et al., 2015) publication. See Fig. S1 for data accumulation and length resolved by CH4 flux type.
3.2 Spatial and temporal distribution of data
Spatially, 40 % of all sites and 52 % of all CH4 concentration observations are in North America, followed by Europe (25 % of all sites and 26 % of all CH4 concentration values; Table S2). Conversely, there are vast geographic areas with moderate to high channel densities with few or no observations, such as central Canada, central America, South America beyond the Amazon mainstem area, most of Russia, central and western Asia, New Zealand, and the Malay Archipelago (Fig. 7a). Geographic limitations in availability of flux data, particularly of ebullition, are pronounced given a smaller number of observations and domination of diffusion measurements. Observations of ebullition are absent or limited to one to two studies for Africa, Oceania, central America, South America, and Russia (Fig. S2). Despite these gaps, there is surprisingly good representation in terms of the range of hydrological, physiographical, climatic, land cover, geological, edaphic, and anthropogenic conditions that exist globally (Fig. 7b). Areas that are poorly represented are characterized by very low channel density associated with arid or polar climates as well as high-altitude regions (Greenland, northern Canada, northern Africa, central Australia, Middle Eastern nations, western China, Mongolia, Chile, southern Argentina). Evaluating the distribution or representativeness of sites in terms of system size is difficult given the limited availability of relevant information such as Strahler stream order or basin area, which were reported for only 26 % and 28 %, respectively, of all sites (Table S2). For sites with these data, counts of observations decline with increasing stream order (Fig. 8) in a log-linear fashion (R2=0.92 for concentration and 0.90 for flux; P<0.0005 for both regressions after excluding zero-order counts), consistent with Horton's law of stream numbers (Horton, 1945). Thus, other than the extreme underrepresentation of zero-order channels, this predictable decline suggests reasonable representation by order. Nonetheless, this result should be interpreted with caution given the scarcity of relevant data. The distribution of counts by basin size follows a similar pattern of underrepresentation of sites draining very small basins and also indicates a potential overrepresentation of some large basin sizes (basins of ca. 10 000 km2; Fig. 8).
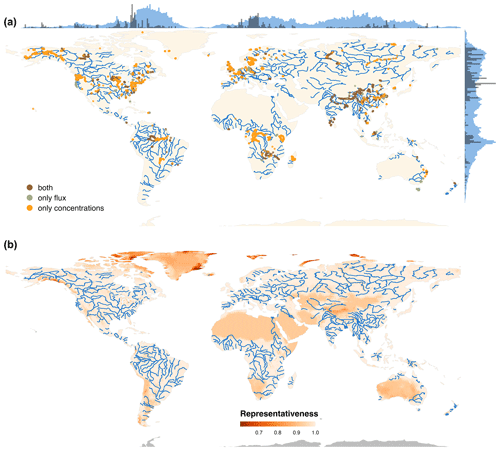
Figure 7(a) Global distribution of methane (CH4) observations in the database, color coded for sites with concentration data only, flux data only, or both concentration and flux data. Top and right panels show, respectively, longitudinal and latitudinal patterns of the density of CH4 observations (grey bars) and the density of river area (blue bars). These bars have been aggregated at a 1 latitudinal or longitudinal degree and rescaled from 0 to 1 for this visualization. River area was obtained from BasinAtlas (Linke et al., 2019). (b) Representativeness (dimensionless) of the database was based on a wide array of biological, physical, hydrological, and land cover variables (see Sect. 2.4 for details). Values close to 1 indicate a high representativeness, with only 4 % of the global river surface below a threshold of 0.9. See Fig. S2 for the data distribution resolved by CH4 flux type.
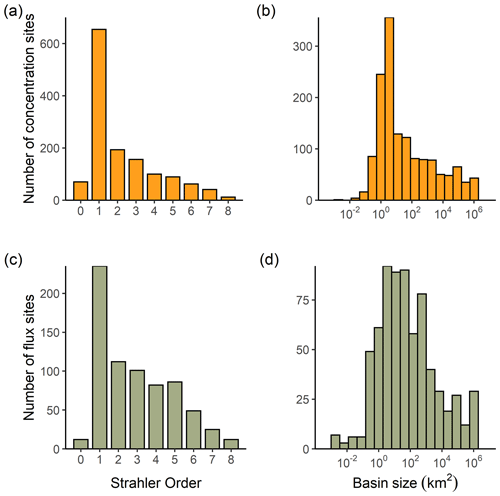
Figure 8Number of sites with concentration (a, b) or diffusive flux (c, d) observations as a function of stream order (a, c) and basin size (b, d) for the subset of sites with channel order and/or basin size information.
The distribution of observations among months illustrates seasonal sampling regimes dominated by summer sampling at northern (>40∘) and southern (∘) latitudes contrasted by even or erratic sampling at mid latitudes (Fig. 9). Consistent with the lower representation of Southern Hemisphere rivers and streams, several months lack concentration and/or flux measurements south of −10∘ latitude, particularly during winter months. Beyond these gaps, the only months missing data in the Northern Hemisphere are fluxes in January and February at sites north of 60∘ latitude and several missing months north of 70∘, presumably due to pervasive ice and snow cover.
3.3 CH4 flux methodology
Records of CH4 flux are dominated by diffusive flux measurements, which represent 85 % of all flux values in the database, with ebullition (8 %) and total flux (7 %) accounting for the remaining entries (Fig. 10). Not surprisingly, a variety of methods have been used to quantify each flux type, although diffusive flux methods are dominated by calculations based on dissolved gas concentration and a gas exchange coefficient (k) (74 % of all observations), while chamber-based methods are most common for quantifying total flux (93 % of all observations). Similarly, k is most commonly estimated via physical models (n = 3188). Several models have been employed for this calculation, as indicated by >25 different references for k model sources listed in GRiMeDB.
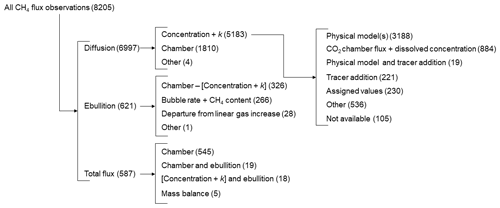
Figure 10Counts of methane (CH4) flux observations by type (left) by major methodological categories for each pathway (middle) and for the method type used to estimate the gas exchange coefficient k (right). For clarity, the chamber category includes all chamber types and patterns of gas increase in the chamber unless specified; more resolved methodological data are presented in the GRiMeDB flux table. See Table A4 for further details about category definitions.
Table 2Summary statistics for methane (CH4), carbon dioxide (CO2), and nitrous oxide (N2O) concentrations and fluxes. The %BDL (below detection level) column reports the percent of all observations that are below detection limits (including values reported as zero) for concentration. See Table S2 for counts and Table S3 for statistical summaries for all other variables. Standard deviation (SD) and coefficient of variation (CV).
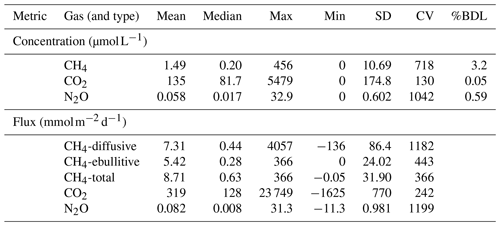
3.4 Overview of concentration and flux data
Concentrations and fluxes of all three gases are characterized by log-normal distributions that vary over several orders of magnitude (Fig. 11) and large coefficients of variation (CVs) for CH4 and especially N2O (Table 2). The vast majority (∼ 95 %) of CH4 and CO2 concentrations appear to be supersaturated, in contrast to N2O concentrations, with 67 % of observations above this threshold. Reports of concentrations below detection are scarce for all gases, including N2O (Table 2).
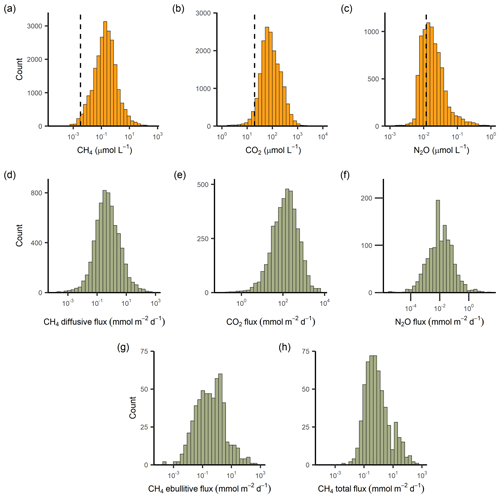
Figure 11Histograms of gas concentrations and fluxes in GRiMeDB, excluding values reported as below detection or zero. Counts of these values are reported in Table S2. Dashed vertical lines in the concentration histograms indicate the 100 % saturation concentration based on the median estimated elevation (250 m) and water temperature (12.5) for all sites and atmospheric concentrations of 1.83, 400, and 0.325 ppm for methane (CH4), carbon dioxide (CO2), and nitrous oxide (N2O), respectively.
The fraction of observations with zero, below-detection, or negative fluxes (5 %, 5 %, and 19 % for diffusive CH4, CO2, and N2O fluxes, respectively) were similar to the frequency of subsaturated concentrations. At the other extreme, the highest CH4 concentrations (> 200 µmol L−1) paradoxically occur in either anthropogenically influenced large rivers of the warm tropics (e.g., Amazon basin: Kemenes et al., 2007; Ganges, Mekong: Begum et al., 2021) or in small boreal headwater streams (e.g., Campeau et al., 2018; Wallin et al., 2018).
There were no meaningful univariate relationships between variables that may be used for upscaling (latitude, basin area, and stream order) and mean site concentration or flux (Fig. 12, Table S3). Linear regressions indicated that latitude and flux accounted for a very small percent of the variation in both concentration (R2=0.006 and 0.002, respectively) and flux (R2 = 0.036 and 0.055) among sites. Similarly, concentration and flux among stream orders suggested possible differences for concentration (Kruskal–Wallis χ2=47.165, df =8, P<0.001) and flux (χ2=14.777, df =8, P=0.070). However, results of corrected pairwise comparisons (using the method of Benjamini and Hochberg, 1995) among orders were ambiguous, suggesting no differences among orders for flux. For concentration, these comparisons indicated possible differences in distributions only between seventh-order channels and all other orders and between sixth- vs. first-order sites for concentration. Collectively, these results indicate a lack of a consistent change in CH4 magnitude across channel orders for flux.
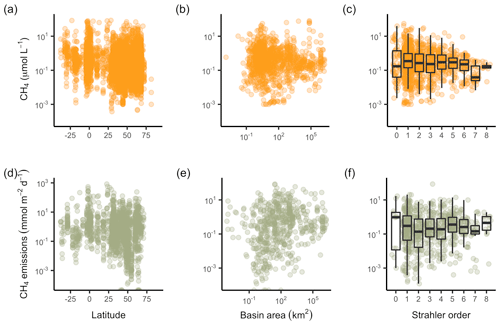
Figure 12Site-average methane (CH4) concentrations (a–c) and flux (d–f) as a function of latitude, basin area, and Strahler stream order. For boxplots, the upper and lower edges of each box are the 25th and 75th percentiles, and whiskers are drawn up to 1.5 times the interquartile range.
As with relationships between CH4 and physical site attributes, relationships between CH4 concentration or flux and water chemistry parameters are also characterized by substantial variability. Representative examples indicate increasing, decreasing, and ambiguous relationships between CH4 concentrations and fluxes and selected chemical constituents (Fig. 13). One source of the variation in the relationship shown in Fig. 13 may be attributed to differences among sites, as is illustrated for the case of CH4 concentration versus discharge. The cluster of points in this plot (Fig. 14a) does not suggest an obvious linear relationship between concentration and discharge; however, resolving the data to the site level for sites with multiple observations reveals several significant trends (Fig. 14b). Among 57 sites with > 30 observations, 42 had significant relationships (P<0.05) between concentration and discharge, and 30 of these 42 trends were negative.
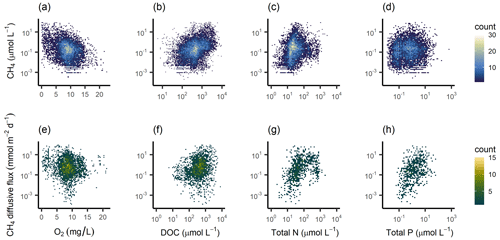
Figure 13Methane (CH4) concentration (a–d) and diffusive flux (e–h) versus concurrent measures of dissolved oxygen (O2; n= 8529 and 2316 for concentration and flux, respectively), dissolved organic carbon (DOC; n= 14 441 and 1901), total nitrogen (total N; n= 8378 and 467), and total phosphorus (total P; n= 6904 and 240). Three outliers were excluded from the DOC plots, and because of the log scale for CH4, negative and zero values have been omitted.
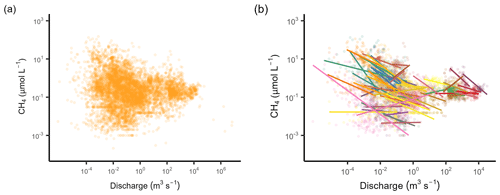
Figure 14Methane (CH4) concentration versus concurrent measures of discharge for (a) all sites with discharge data and (b) sites with > 30 observations (57 sites) with trend lines denoting within-site relationships between concentration and discharge. Each site is represented by a separate color. Because of the log scale for CH4, negative and zero values are omitted.
Median site concentrations for most categories of targeted channels (Fig. 15) differed from “normal” (NORM) sites (Kruskal–Wallis test χ2 =460.1, df =12, P<0.0001 after dropping channel types with <10 observations to improve test reliability). Pairwise Wilcoxon comparisons adjusted to account for multiple comparisons (Benjamini and Hochberg, 1995) indicated that springs (SP) and delta channels (DC) were similar to NORM sites (P>0.4), and impoundment-influence (IMP) sites were marginally different (P=0.053). Concentrations in channels at glacial termini (GT) and FP backwaters were lower (P<0.0001), whereas all other site types had higher site-average CH4 concentrations than NORM sites. Fluxes also varied among channel type (Kruskal–Wallis test χ2=126.4, df = 8, P<0.0001 after dropping channel types with <10 observations), and similar to concentration, fluxes in DC and channelized sites (CH) were similar to NORM channels, while all other channel types considered had higher median fluxes.
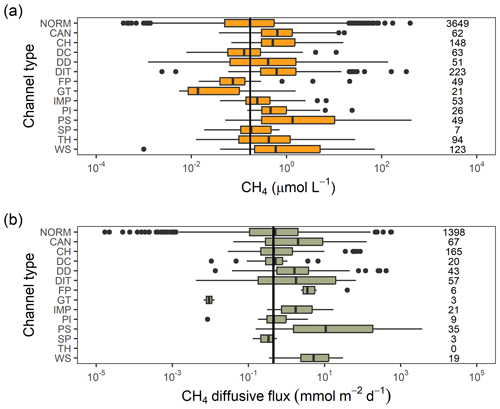
Figure 15Boxplots of site-averaged methane (CH4) concentration (a) and diffusive flux (b) for channel-type categories. Channel categories are defined in Table 1 but briefly are as follows: NORM – non-targeted sites; CAN – canals; CH – channelized streams; DC – river delta channels; DD – downstream of dams; DIT – ditches; FP – floodplain backwaters; GT – glacial outflows; IMP – impounded reaches; PI – permafrost (thermokarst) influenced; PS – point source influenced; SP – springs; TH – thermogenic CH4 inputs; WS – wetland streams. The number of sites per channel type are listed on the right-hand side of each plot. The vertical black line denotes the median concentration and flux for NORM sites. Because a log scale is used in these plots, zeros and negative values were excluded. The actual median for non-targeted sites represented by the vertical line is therefore slightly different than the median displayed in the corresponding boxplot because of this exclusion. The upper and lower edges of each box are the 25th and 75th percentiles, whiskers are drawn up to 1.5 times the interquartile range, and points are plotted if beyond the whiskers.
The rapid increase in availability of aquatic CH4 (as well as CO2 and N2O) data over the past 5–10 years has been remarkable and creates new opportunities for examining patterns and drivers of these gases in lotic ecosystems across broad spatial scales. Similarly, constructing GRiMeDB provided us with an unprecedented opportunity to identify tendencies in when, where, and how CH4 has been sampled in streams and rivers. Examination of such data collection tendencies can reveal important biases and gaps within a field (Stanley et al., 2019; Gomez-Gener et al., 2021b) and thus points to future research needs and opportunities. Below, we discuss the distribution of sampling efforts, methodological issues, and preliminary data analyses and consider questions that GRiMeDB can help to answer.
4.1 When and where: sampling effort considerations
The growth of greenhouse gas (GHG) studies in flowing water systems in the past decade includes a geographic expansion beyond the large body of historic and current work in temperate regions of North America and Europe. In particular, recent research in Africa, Australia, and especially Southeast Asia has greatly improved the global coverage of available data. However, studies in arid drainages remain scarce – even beyond what would be expected given their small river surface area. A possible explanation for the limited study of CH4 in these systems may be the pervasive focus on the contribution of streams and rivers to the global atmospheric CH4 pool and the corresponding assumption that aridland systems play a minor role in this context. However, we suggest that limited study in arid and semi-arid drainages represents a missed opportunity to understand metabolism and carbon cycling in a set of streams and rivers that drain nearly half of the global land surface, is increasingly stressed by growing human water demands (e.g., Sabo et al., 2010; Lian et al., 2021; Stringer et al., 2021), and supports ecosystem process rates that are amplified by warm temperatures and highly variable flow regimes (Fisher et al., 1982; Ran et al., 2021). Beyond arid and semi-arid basins, further research emphasis in tropical and high-latitude regions would also be beneficial even given recent improvements in data availability and geographic representation of both areas. Existing data for tropical forests and grasslands are dominated by studies of African rivers (especially the Congo drainage) and the Amazon River system. In fact, observations from tropical areas of the Indomalayan and northern Australasian region represent < 3 % of all sites, and central America is represented by a single study. Tropical drainages are frequently characterized by high CH4 concentrations and fluxes along with rapid changes in land use and river regulation that are affecting C cycling and GHG dynamics (Park et al., 2018; Flecker et al., 2022). However, understanding or detecting the magnitude and consequences of these anthropogenic changes in fluvial CH4 is constrained by these current sampling limitations. Finally, while high-latitude regions (north of the Arctic Circle) are well represented in GriMeDB with > 3600 concentration observations, more than 80 % of these values are derived from studies in the vicinity of the Toolik Field Station in Alaska, USA, and thus do not capture the full biophysical diversity of Arctic biomes (Metcalfe et al., 2018). Given that climate change at high latitudes is progressing faster than elsewhere on the planet (IPCC, 2021) and that the global north stores massive quantities of C in soils (Hugelius et al., 2014), more extensive coverage of CH4 across Arctic drainage systems is warranted.
Although the spatial coverage of CH4 data has improved markedly over the past decade, expansion across temporal dimensions has lagged. The predominant mode of sample collection has been and continues to be through surveys that yield one or a few observations from individual sites (e.g., Bouillon et al., 2012; Kuhn et al., 2017; Jin et al., 2018; Ho et al., 2022), and studies characterizing seasonal dynamics or responses to a site-specific environmental change are limited. Indeed, long-term (> 5-year) CH4 data sets in general are extremely rare (Leng et al., 2021); no such data are currently available for fluxes, and most long-term concentration records are derived from just a few clustered locations. Determining the consequences of changes in land use or habitat attributes for fluvial CH4 dynamics has instead relied on space-for-time substitutions (e.g., Smith et al., 2017; Gatti et al., 2018; Woda et al., 2020) rather than on direct observations of change over time. Although this strategy has been successful in revealing variation in GHG dynamics among different site types, current knowledge about how gases vary over time and respond to perturbations is poorly developed because of these data limitations. This deficit may be particularly consequential in the case of climate change, as the broad scope of this phenomenon will inevitably limit the effectiveness of spatial sampling approaches.
The discussion above regarding the “when” and “where” of sampling emphasizes large spatial and relatively long temporal scales, consistent with the extent of GRiMeDB. However, another current deficit in our understanding relates to the degree of heterogeneity of this gas at fine spatial and temporal scales and thus whether current sampling strategies are missing meaningful variation. Recent studies of CO2 provide a cautionary tale in this context, as failure to account for diurnal variation in this gas results in a consistent underestimation of fluvial emissions that is quantifiable at regional (Attermeyer et al., 2021) and global (Gómez-Gener et al., 2021b) scales. Similar questions may arise for spatial variation: that is, what is the minimum grain size or appropriate spatial scale for sampling CH4 in running waters (Crawford et al., 2017; Lupon et al., 2019)? The potential to examine very short-term variation is not possible using GRiMeDB data because of our decision to average within-day measurements given the current small number (ca. 20) of these temporally detailed studies. Assessment of fine-scale spatial variation is also limited because of limited fine-scale sampling in general and by decisions made both by investigators and during database construction. For example, geomorphologically distinct units (e.g., an individual riffle or pool) are often used as a basic sampling unit, and results are presented as averages of replicates collected at different points within the study reach (e.g., Hlaváčová et al., 2006; Smith et al., 2017). In general, information about replication was frequently omitted, or if reported, information about variability among replicates was frequently absent. In addition to this limitation, our decision to combine replicates taken at different points in a channel cross section and within individual channel units that had hundreds to thousands of data points to avoid ambiguities for site delineation and data analysis also constrains the opportunity to examine variation at fine spatial scales. However, we anticipate that this situation will change over the next few years as in situ sensors or other devices capable of collecting high-frequency/high-density gas measurements become more widely available. Recent papers signal this new frontier and have highlighted the presence (e.g., Lamarche-Gagnon et al., 2019; Smith and Böhlke, 2019; Chen et al., 2021; Taillardat et al., 2022) and absence (e.g., Castro-Morales et al., 2022; Chen et al., 2021; Rovelli et al., 2022; Zhang et al., 2021) of predictable diel variation in CH4 concentrations and fluxes and varying degrees of within-reach spatial variability (Crawford et al., 2016; 2017; Call et al., 2018; Bussmann et al., 2022).
4.2 How: methodological considerations
Measuring dissolved GHG concentrations or fluxes involves multiple steps and calculations. Field and laboratory protocols vary widely in the literature, and methodological variety is particularly conspicuous for flux determination. Ironically, even though many studies of lotic CH4 dynamics are framed in terms of understanding the contribution of these ecosystems to the rapidly increasing atmospheric CH4 pool, flux measurements lag far behind those of concentrations, and the vast majority (ca. 85 %) of observations are of the diffusive pathway alone. Further, the most common method for estimating this pathway involves combining dissolved CH4 concentration with k, the gas exchange coefficient. Quantifying k is notoriously challenging (Hall and Ulseth, 2020), and the large number of approaches for calculating k used among data providers is concerning and undoubtedly introduces substantial uncertainty. A more in-depth consideration of the consequences of different models or strategies for arriving at a k value was beyond the scope of this paper, but inclusion of methodological information should be useful for such an analysis in the future.
Ebullition measurements are notably scarce despite the potential of this pathway to account for a large fraction of total emissions in some streams (e.g., from 30 % to 98 % of total CH4 emissions, as shown in Baulch et al., 2011; Crawford et al., 2014; Chen et al., 2021). The conventional approach to quantifying ebullition involves a combination of capturing bubbles just below the water surface to determine the area and time-specific rate of bubble volume reaching the surface and measuring CH4 content of recently erupted bubbles. The episodic nature and extreme spatial heterogeneity of ebullition (Crawford et al., 2014; Spawn et al., 2015; Chen et al., 2021; Robison et al., 2021) require multiple bubble trap replicates that need to be deployed over several days to generate reliable measurements. Given the logistic challenges and labor-intensive work involved, indirect approaches are becoming more common. These approaches typically use the difference between a chamber-based measurement of flux, which is assumed to represent total flux (diffusion + ebullition) and diffusion calculated from dissolved CH4 and k (i.e., the “chamber – [concentration + k]” method in Fig. 10) to estimate ebullition (e.g., Campeau et al., 2014; Zhang et al., 2020; Ran et al., 2021). We suggest that this approach should be used cautiously, however. This strategy is arguably inappropriate for situations in which the gas content within a chamber increases in a linear fashion during the measurement period, consistent with the occurrence of diffusive flux alone. Second, it is not clear whether it is reasonable to assume that chamber-based measurements capture both diffusion and ebullition, even if a chamber-based flux value is greater than that calculated from a dissolved CH4 concentration. Relatively short chamber deployments are likely to miss or incompletely capture bubble releases, while long-term deployments are vulnerable to sampling artifacts associated with altered concentration gradients within and/or turbulence around the chamber (Sawakuchi et al., 2014; Lorke et al., 2015). Given these challenges, it is not altogether surprising that comparisons between direct and indirect measurements of ebullition can yield substantially different results (e.g., Yang et al., 2012; Bednařík et al., 2017; Chen et al., 2021).
The final and most profound knowledge gap in the collection of flux data is the absence of measurements of plant-mediated emissions. Plant-mediated fluxes can account for a substantial fraction of total emissions from wetlands and shallow lake habitats (Bodmer et al., 2021), but the contribution of this pathway is unknown in fluvial systems. Indeed, we did not include plant-mediated fluxes in GRiMeDB because we encountered only two papers that had explicitly quantified this pathway in streams (Sanders et al., 2007; Wilcock and Sorrell, 2008). Although aquatic macrophytes are sparse or absent from many streams and rivers, they can be abundant in low-gradient, low-disturbance environments (Riis and Biggs, 2003; Gurnell et al., 2010), where diffusive fluxes would be constrained by low gas exchange rates. Sediment trapping and venting by macrophytes enhance both methanogenesis and methane emission in these systems (Sanders et al., 2007), but the significance of such processes and the contribution of plant-mediated fluxes at larger spatial scales remain to be determined for fluvial systems (Bodmer et al., 2021).
4.3 Concentration and flux patterns
Not surprisingly, the massive increase in data availability has led to differences in averages and measures of variability for CH4 concentrations and fluxes compared to our previous efforts. Median values for all three CH4 flux pathways in GRiMeDB are 1.2–2.2 times lower than those reported by Stanley et al. (2016) and those from Rosentreter et al. (2021). Conversely, measures of variability (SD, CV) in GRiMeDB are almost 3-fold greater than previous estimates, undoubtedly due to the far larger number of observations, the associated expansion of geographic scope and channel types, and the inclusion of temporally resolved data. It is not yet clear whether the sample sizes are sufficient to capture the true global-scale variability of fluvial concentrations and fluxes, and future database updates could be used to examine this possibility.
Despite the slight lowering of median values compared to previous estimates, supersaturated concentrations and positive fluxes are the norm for CH4 as well as for CO2 and N2O. However, it is likely that CH4 concentrations and flux BDLs are underreported, as is common with environmental data in general (Stow et al., 2018), so these latest estimates may still be slight overestimations of true population medians. Even given the modest number of zero or undetectable CH4 concentrations in GriMeDB (< 2.5 %), decisions about handling BDLs can have a small but detectable effect on the estimation of global averages. For example, if these observations are excluded, median CH4 concentrations for all other observations increase from 1.49 to 1.51 µmol L−1. If we keep all of these observations and assign them a value of zero (an unlikely scenario but used here to provide a lower limit for this example), then the overall median declines to 1.46 µmol L−1. Although these differences are relatively small, it would likely be consequential for upscaling estimates. At a minimum, we urge GRiMeDB users to be aware of how these values are handled and encourage future researchers to determine and report detection limits and include samples that fall below these limits in their results.
A goal of assembling GRiMeDB was to centralize CH4 data to foster future research efforts. To this end, we included information about habitat conditions that allows the exploration of relationships between CH4 and potential explanatory variables and covariates. To demonstrate this opportunity, we provided a limited number of examples of CH4 versus variables that have been identified as potential predictors or drivers of CH4 production, concentration, or flux (Figs. 12–14), and these plots suggest both the presence and absence of relationships. For example, increasing CH4 concentrations have been associated with low or decreasing dissolved oxygen and/or increasing organic carbon (e.g., Borges et al., 2018; Jin et al., 2018; Begum et al., 2021), and these relationships are recognizable for concentration but ambiguous for flux across the entirety of the GRiMeDB data set. Similarly, increased CH4 production and emissions tend to be elevated in nutrient-rich (eutrophic) lakes (DelSontro et al., 2018) and polluted rivers (Rajkumar et al., 2008; Ho et al., 2022), consistent with positive relationships between CH4 flux and total nitrogen (TN) and total phosphorus (TP). However, nutrient enrichment in rivers often occurs concurrently with fine sediment and organic matter input; thus, it remains to be determined whether positive relationships in Fig. 13g and h are correlative or reflect a causal mechanism. Finally, increases in discharge have been linked to declines in gas concentration, likely due to source limitation (i.e., dilution) of terrestrial supply (Aho et al., 2021; Gómez-Gener et al., 2021a) and/or greater water turbulence, which increases gas exchange and thus reduces supersaturated CH4 stocks (Billett and Harvey, 2013; Kokic et al., 2018). This relationship is not obvious when all data were considered en masse but became more apparent when examining within-site dynamics. In contrast to these three confirmatory examples, although latitude and channel size have also been identified as determinants of CH4 concentrations or used to extrapolate site-specific gas measurements to larger (even global) scales (e.g., Bastviken et al., 2011; Li et al., 2021; Rosentreter et al., 2021), evidence for such relationships is absent from our analysis. Further, even for the former examples that indicated relationships between CH4 concentration and dissolved oxygen (DO), dissolved organic carbon (DOC), or discharge, there is substantial variability present in these relationships, the strength of these predictors is likely to vary across scales, and they explain little of the variability for diffusive fluxes. In short, substantial opportunities exist to identify multivariate relationships between different predictors and CH4 concentrations and fluxes across different scales, and pursuit of these opportunities should be improved by the substantial increase in data for both gases and potential predictor variables.
The disproportionate contribution of streams and rivers to atmospheric inputs and the utility of CH4 as an indicator of anthropogenic influences on drainage systems have inspired several studies that focus on fluvial habitats that are expected to have high concentrations and fluxes. Many of these “methane-hunting” studies have demonstrated significant increases in CH4 concentrations and/or fluxes associated with phenomena such as point source inputs (Alshboul et al., 2016), ditch and canal construction (Peacock et al., 2021), oil and gas extraction (Woda et al., 2020), or passage through wetlands (Taillardat et al., 2022). Such signals persist at the global scale (Fig. 15), highlighting widespread human enhancement of CH4 emissions from lotic ecosystems. Not all targeted sites are CH4-rich, however. Low concentrations in GT likely reflect the effects of cold temperatures and/or low organic carbon availability (Crawford et al., 2015; Burns et al., 2018), while low values at FP sites may be attributable to their more characteristically lentic conditions, which favor higher rates of CH4 oxidation in the water column. Indeed, oxidation has been shown to represent a significant CH4 sink in floodplain lakes associated with the Amazon River (Barbosa et al., 2018), and most of the FP sites in GRiMeDB are part of the Amazon system.
As noted in Sect. 3.4, the availability of supporting information is inconsistent, as, for example, only ∼ 25 % of data sources provided data on channel order or basin size. However, open-access regional and global geospatial data sets that provide information about site characteristics (e.g., Linke et al., 2019; Yang et al., 2020) have increased rapidly in the past decade, to the benefit of analyses seeking to link landscape attributes to CH4 distribution among sites. Recent upscaling effort analyses (Rosentreter et al., 2021; Liu et al., 2022; Rocher-Ros et al., 2023) have, for example, capitalized on improved estimates of the surface area of world streams and rivers (Allen and Pavelsky, 2018; Yang et al., 2020), while the diverse data sets in HydroSHEDS (Linke et al., 2019) allowed us to evaluate the global representativeness of GRiMeDB sites. As new global-scale data sets become available and become more spatially resolved, we anticipate that their pairing with GRiMeDB data will result in significant improvements in the strength and certainty of data-assimilation models, regional- to global-scale analyses of CH4 distribution and drivers, and quantification of fluvial emissions into the atmosphere.
GRiMeDB and its associated metadata are available from the Environmental Data Initiative: https://doi.org/10.6073/pasta/f48cdb77282598052349e969920356ef (Stanley et al., 2023).
The data gathered in GRiMeDB highlight many new opportunities, both through analysis of CH4 and supporting data in the database and by revealing gaps that currently exist across fluvial CH4 studies. The most conspicuous data limitations include deficits in measurements of non-diffusive flux pathways and underrepresentation of sites from arid, tropical, and arctic biomes. Challenges associated with quantifying ebullition discussed earlier also emphasize the need for more intercomparisons among flux methodologies. Regardless of pathway, flux is a difficult process to quantify and can be highly sensitive to methods or gas exchange model choices, yet there are few comparisons (such as Raymond et al., 2012; Lorke et al., 2015) available to inform these decisions. Finally, we highlight that the expansion of GHG data over the past decade has proceeded largely across spatial rather than temporal dimensions. While this expansion has vastly improved the geographic representativeness of the data, long-term data sets are rare despite their power for generating ecological understanding and informing policy/management in the face of environmental change (Hughes et al., 2017). GHGs are rarely included as routine components of water quality monitoring programs. Thus, we emphasize the compelling need to establish such sampling efforts and perpetuate those few that do exist.
Given the rapid growth in both research interest and data in fluvial GHG dynamics, we imagine future updates and expansion of GRiMeDB, and we welcome data sets and associated research products (e.g., theses, journal publications, reports). To facilitate the data acquisition and updating process, a downloadable spreadsheet template and detailed information about its use and submission are available at https://stanley.limnology.wisc.edu/GRiMe (last access: 12 June 2023). Regardless of database updates, we recommend that the minimum basic information to collect along with GHG data that would be most valuable for later analyses include well-resolved site location data (latitude and longitude), information about site size (Strahler order and/or basin size at the sampling site), disturbance or modification relevant to GHGs (e.g., categories listed in Table 1), specific sample dates and times, discharge, dissolved oxygen, and temperature at the time of sample collection, and clear information about units and method(s) used to measure gas flux. Finally, we strongly encourage data package (sensu Gries et al., 2022) publication in a trustworthy public data repository such as the Environmental Data Initiative that requires metadata to meet findability, accessibility, interoperability, and reusability (FAIR) data principles and increase data findability, accessibility, and reuse (Wilkinson et al., 2016).
Despite highlighting areas of data limitation, it is important to underscore the opportunities that the growth in GHG data availability – especially of CH4 data – now provides. Assembly of GRiMeDB was motivated by the goal of having a centralized, standardized resource to facilitate further studies of CH4 pattern and process in flowing water systems. Our strategy in developing this database was to maximize opportunities for identifying patterns and relationships involving this gas in future analyses. Past difficulties with such efforts may well be a product of the common practice of averaging values over time or among sites and/or of including non-fluvial sites in analyses. Thus, we carefully documented the data and resolved observations to individual sites and dates whenever possible to match the pronounced spatial and temporal variance of this gas. Similarly, while we included a range of habitat types in GRiMeDB, unconventional or targeted sites are easily identifiable. Further, we carefully examined sites to ensure that they were not situated within reservoirs/impoundments or estuaries where distinct processes such as methane oxidation, tidal cycles, or elevated sulfate reduction may obscure or overtake relationships present in inland-flowing water systems. Thus, we are optimistic that analysis of GriMeDB data by themselves or in concert with other complementary data sets will provide new and unprecedented opportunities to examine relationships between CH4 and environmental drivers or correlates and provide broad contextual information for site-based studies of fluvial carbon and GHG dynamics.
Abbott, B. and Jones, J.: Soil respiration, water chemistry, and soil gas data for thermokarst features and undisturbed tundra on the North Slope of Alaska, Arctic Data Center, https://doi.org/10.18739/A23T9D71C, 2013.
Abbott, B. W., Jones, J. B., Godsey, S. E., Larouche, J. R., and Bowden, W. B.: Patterns and persistence of hydrologic carbon and nutrient export from collapsing upland permafrost, Biogeosciences, 12, 3725–3740, https://doi.org/10.5194/bg-12-3725-2015, 2015.
Abril, G., Guérin, F., Richard, S., Delmas, R., Galy-Lacaux, C., Gosse, P., Tremblay, A., Varfalvy, L., Dos Santos, M. A., and Matvienko, B.: Carbon dioxide and methane emissions and the carbon budget of a 10-year old tropical reservoir (Petit Saut, French Guiana), Global Biogeochem. Cy., 19, GB4007, https://doi.org/10.1029/2005GB002457, 2005.
Adams, D. D. and Simiyu, G. M.: Greenhouse gas (methane and carbon dioxide) emissions from a tropical river in Kenya: the importance of anthropogenic factors on natural gas flux rates, SIL Proceedings 1922–2010, 30, 887–889, https://doi.org/10.1080/03680770.2009.11902264, 2009.
Aho, K., Cawley, K., DelVecchia, A., Stanley, E., and Raymond, P.: Dissolved greenhouse gas concentrations derived from the NEON dissolved gases in surface water data product (DP1.20097.001), Environmental Data Initiative, https://doi.org/10.6073/pasta/47d7cb6d374b6662cce98e42122169f8, 2021a
Aho, K., Fair, J., Hosen, J., Kyzivat, E., Logozzo, L., Weber, L., Yoon, B., Zarnetske, J., and Raymond, P.: Dissolved N2O measurements from the Connecticut River Watershed, Environmental Data Initiative, https://doi.org/10.6073/pasta/3494ca49fc3283eea5e4fc2f8a24ce3b, 2021c.
Aho, K., Hoyle, J., Hosen, J., Kyzivat, E., Logozzo, L., Rocher-Ros, G., Weber, L., Bryan, Y., and Raymond, P.: Dissolved CO2 and CH4 concentrations in the Connecticut River Watershed, Environmental Data Initiative, https://doi.org/10.6073/pasta/af4daec813775b7f426a1db574cbebc7, 2021d.
Aho, K. S. and Raymond, P. A.: Differential response of greenhouse gas evasion to storms in forested and wetland streams, J. Geophys. Res.-Biogeo., 124, 649–662, https://doi.org/10.1029/2018JG004750, 2019.
Aho, K. S., Fair, J. H., Hosen, J. D., Kyzivat, E. D., Logozzo, L. A., Rocher-Ros, G., Weber, L. C., Yoon, B., and Raymond, P. A.: Distinct concentration-discharge dynamics in temperate streams and rivers: CO2 exhibits chemostasis while CH4 exhibits source limitation due to temperature control, Limnol. Oceanogr., 66, 3656–3668, https://doi.org/10.1002/lno.11906, 2021b.
Alshboul, Z., Encinas-Fernández, J., Hofmann, H., and Lorke, A.: Export of dissolved methane and carbon dioxide with effluents from municipal wastewater treatment plants, Environ. Sci. Technol., 50, 5555–5563, https://doi.org/10.1021/acs.est.5b04923, 2016.
Andrews, L. F., Wadnerkar, P. D., White, S. A., Chen, X., Correa, R. E., Jeffrey, L. C., and Santos, I. R.: Hydrological, geochemical and land use drivers of greenhouse gas dynamics in eleven sub-tropical streams, Aquat. Sci., 83, 40, https://doi.org/10.1007/s00027-021-00791-x, 2021.
Anthony, S. E., Prahl, F. G., and Peterson, T. D.: Methane dynamics in the Willamette River, Oregon, Limnol. Oceanogr., 57, 1517–1530, https://doi.org/10.4319/lo.2012.57.5.1517, 2012.
Antweiler, R. C., Smith, R. L., Voytek, M. A., Bohlke, J. K., and Dupré, D. H.: Water quality data from two agricultural drainage basins in Northwestern Indiana and northeastern Illinois: III. Biweekly data, 2000–2002, U.S. Geological Survey, https://pubs.usgs.gov/of/2005/1197/ (last access: 12 June 2023), 2005a.
Antweiler, R. C., Smith, R. L., Voytek, M. A., Böhlke, J.-K., and Richards, K. D.: Water quality data from two agricultural drainage basins in northwestern Indiana and northeastern Illinois: I. Lagrangian and synoptic data, 1999–2002, U.S. Geological Survey, http://pubs.water.usgs.gov/ofr20041317/ (last access: 12 June 2023), 2005b.
Arp, C., Kane, D., Hinzman, L., and Stuefer, S.: Hydrographic data, Imnavait Creek Watershed, Alaska, 1985–2017, Arctic Data Center, https://doi.org/10.18739/A2K649S9D, 2017.
Atkins, M. L., Santos, I. R., and Maher, D. T.: Seasonal exports and drivers of dissolved inorganic and organic carbon, carbon dioxide, methane and δ13C signatures in a subtropical river network, Sci. Total Environ., 575, 545–563, https://doi.org/10.1016/j.scitotenv.2016.09.020, 2017.
Audet, J., Wallin, M. B., Kyllmar, K., Andersson, S., and Bishop, K.: Nitrous oxide emissions from streams in a Swedish agricultural catchment, Agr. Ecosyst. Environ., 236, 295–303, https://doi.org/10.1016/j.agee.2016.12.012, 2017.
Bagnoud, A., Pramateftaki, P., Bogard, M. J., Battin, T. J., and Peter, H.: Microbial ecology of methanotrophy in streams along a gradient of CH4 availability, Front. Microbiol., 11, 771, https://doi.org/10.3389/fmicb.2020.00771, 2020.
Baker, M. A., Dahm, C. N., Valett, H. M., Morrice, J. A., Henry, K. S., Campana, M. E., and Wroblicky, G. J.: Spatial and temporal variation in methane distribution at the ground water/surface water interface in headwater catchments, in: 2nd International Conference on Ground Water Ecology, Atlanta, GA, 27–30 March 1994, 29–37, https://www.osti.gov/biblio/37567 (last access: 12 June 2023), 1994.
Ballester, M. V. R. and dos Santos, J. E.: Biogenic gases in tropical floodplain river, Braz. Arch. Biol. Techn., 44, 141–147, https://doi.org/10.1590/S1516-89132001000200006, 2001.
Bange, H. W., Sim, C. H., Bastian, D., Kallert, J., Kock, A., Mujahid, A., and Müller, M.: Nitrous oxide (N2O) and methane (CH4) in rivers and estuaries of northwestern Borneo, Biogeosciences, 16, 4321–4335, https://doi.org/10.5194/bg-16-4321-2019, 2019.
Banks, E. W., Hatch, M., Smith, S., Underschultz, J., Lamontagne, S., Suckow, A., and Mallants, D.: Multi-tracer and hydrogeophysical investigation of the hydraulic connectivity between coal seam gas formations, shallow groundwater and stream network in a faulted sedimentary basin, J. Hydrol., 578, 124132, https://doi.org/10.1016/j.jhydrol.2019.124132, 2019.
Bansal, S.: PPR stream and river methane, U.S. Geological Survey Northern Prairie Wildlife Research Center, Jamestown, ND, USA, unpublished, 2020.
Barbosa, P. M., Melack, J. M., Farjalla, V. F., Amaral, J. H. F., Scofield, V., and Forsberg, B. R.: Diffusive methane fluxes from Negro, Solimões and Madeira rivers and fringing lakes in the Amazon basin: Diffusive methane fluxes from rivers and fringing lakes, Limnol. Oceanogr., 61, S221–S237, https://doi.org/10.1002/lno.10358, 2016.
Bastien, J. and Demarty, M.: Spatio-temporal variation of gross CO2 and CH4 diffusive emissions from Australian reservoirs and natural aquatic ecosystems, and estimation of net reservoir emissions, Lakes Reservoirs Res. Manage., 18, 115–127, https://doi.org/10.1111/lre.12028, 2013.
Baulch, H. M., Dillon, P. J., Maranger, R., and Schiff, S. L.: Diffusive and ebullitive transport of methane and nitrous oxide from streams: Are bubble-mediated fluxes important?, J. Geophys. Res., 116, G04028, https://doi.org/10.1029/2011JG001656, 2011.
Beaulieu, J. J.: Controls on greenhouse gas emissions from headwater streams, PhD thesis, University of Notre Dame, Notre Dame, IN, USA, 205 pp., https://onesearch.library.nd.edu/permalink/f/1phik6l/ndu_aleph002323859 (last access: 12 June 2023), 2007.
Beaulieu, J. J., Shuster, W. D., and Rebholz, J. A.: Controls on gas transfer velocities in a large river, J. Geophys. Res., 117, G02007, https://doi.org/10.1029/2011JG001794, 2012.
Bednařík, A., Čáp, L., Maier, V., and Rulík, M.: Contribution of methane benthic and atmospheric fluxes of an experimental area (Sitka Stream), CLEAN-Soil Air Water, 43, 1136–1142, https://doi.org/10.1002/clen.201300982, 2015.
Bednařík, A., Blaser, M., Matoušů, A., Hekera, P., and Rulík, M.: Effect of weir impoundments on methane dynamics in a river, Sci. Total Environ., 584–585, 164–174, https://doi.org/10.1016/j.scitotenv.2017.01.163, 2017.
Begum, M. S., Bogard, M. J., Butman, D. E., Chea, E., Kumar, S., Lu, X., Nayna, O. K., Ran, L., Richey, J. E., Tareq, S. M., Xuan, D. T., Yu, R., and Park, J.: Localized pollution impacts on greenhouse gas dynamics in three anthropogenically modified Asian river systems, J. Geophys. Res.-Biogeo., 126, e2020JG006124, https://doi.org/10.1029/2020JG006124, 2021a.
Begum, M. S., Bogard, M. J., Butman, D. E., Chea, E., Kumar, S., Lu, X., Nayna, O., K, Ran, L., Richey, J E, Tareq, S. M, Xuan, D. T., Yu, R., and Park, J.: Spatiotemporal variation in pCO2, CH4, N2O, DOM, and ancillary water quality measured in the Ganges, Mekong, and Yellow River during 2016 to 2019, PANGAEA, https://doi.org/10.1594/PANGAEA.926582, 2021b.
Billett, M. F. and Harvey, F. H.: Measurements of CO2 and CH4 evasion from UK peatland headwater streams, Biogeochemistry, 114, 165–181, https://doi.org/10.1007/s10533-012-9798-9, 2013.
Billett, M. F. and Moore, T. R.: Supersaturation and evasion of CO2 and CH4 in surface waters at Mer Bleue peatland, Canada, Hydrol. Process., 22, 2044–2054, https://doi.org/10.1002/hyp.6805, 2008.
Bilsley, N.: Processes affecting the spatial and temporal variability of methane in a temperate dammed river system, MS thesis, University of California San Diego, San Diego, CA, USA, 94 pp., https://search-library.ucsd.edu/permalink/01UCS_SDI/ld412s/alma991004859049706535 (last access: 12 June 2023), 2012.
Blackburn, S. R. and Stanley, E. H.: Greenhouse gas fluxes and concentrations and associated habitat data in western Dane County, Wisconsin, USA, streams during the 2018 growing season ver. 2., Environmental Data Initiative, https://doi.org/10.6073/pasta/eeba6193162bf1189a6b78452686c773, 2019.
Blackburn, S. R. and Stanley, E. H.: Floods increase carbon dioxide and methane fluxes in agricultural streams, Freshwater Biol., 66, 62–77, https://doi.org/10.1111/fwb.13614, 2021.
Bodmer, P., Heinz, M., Pusch, M., Singer, G., and Premke, K.: Carbon dynamics and their link to dissolved organic matter quality across contrasting stream ecosystems, Sci. Total Environ., 553, 574–586, https://doi.org/10.1016/j.scitotenv.2016.02.095, 2016.
Bolpagni, R., Laini, A., Mutti, T., Viaroli, P., and Bartoli, M.: Connectivity and habitat typology drive CO2 and CH4 fluxes across land-water interfaces in lowland rivers: C fluxes across lowland river interfaces, Ecohydrology, 12, e2036, https://doi.org/10.1002/eco.2036, 2019.
Borges, A. V., Abril, G., Darchambeau, F., Teodoru, C. R., Deborde, J., Vidal, L. O., Lambert, T., and Bouillon, S.: Divergent biophysical controls of aquatic CO2 and CH4 in the World's two largest rivers, Sci. Rep.-UK, 5, 15614, https://doi.org/10.1038/srep15614, 2015a.
Borges, A. V., Darchambeau, F., Teodoru, C. R., Marwick, T. R., Tamooh, F., Geeraert, N., Omengo, F. O., Guérin, F., Lambert, T., Morana, C., Okuku, E., and Bouillon, S.: Globally significant greenhouse-gas emissions from African inland waters, Nat. Geosci., 8, 637–642, https://doi.org/10.1038/ngeo2486, 2015b.
Borges, A. V., Abril, G., and Bouillon, S.: Carbon dynamics and CO2 and CH4 outgassing in the Mekong delta, Biogeosciences, 15, 1093–1114, https://doi.org/10.5194/bg-15-1093-2018, 2018a.
Borges, A. V., Darchambeau, F., Lambert, T., Bouillon, S., Morana, C., Brouyère, S., Hakoun, V., Jurado, A., Tseng, H.-C., Descy, J.-P., and Roland, F. A. E.: Effects of agricultural land use on fluvial carbon dioxide, methane and nitrous oxide concentrations in a large European river, the Meuse (Belgium), Sci. Total Environ., 610–611, 342–355, https://doi.org/10.1016/j.scitotenv.2017.08.047, 2018b.
Borges, A. V. and Bouillon, S.: data-base of CO2, CH4, N2O and ancillary data in the Congo River (latest), Zenodo, https://doi.org/10.5281/ZENODO.3413449, 2019.
Bouillon, S., Abril, G., Borges, A. V., Dehairs, F., Govers, G., Hughes, H. J., Merckx, R., Meysman, F. J. R., Nyunja, J., Osburn, C., and Middelburg, J. J.: Distribution, origin and cycling of carbon in the Tana River (Kenya): a dry season basin-scale survey from headwaters to the delta, Biogeosciences, 6, 2475–2493, https://doi.org/10.5194/bg-6-2475-2009, 2009.
Bouillon, S., Yambélé, A., Spencer, R. G. M., Gillikin, D. P., Hernes, P. J., Six, J., Merckx, R., and Borges, A. V.: Organic matter sources, fluxes and greenhouse gas exchange in the Oubangui River (Congo River basin), Biogeosciences, 9, 2045–2062, https://doi.org/10.5194/bg-9-2045-2012, 2012.
Bouillon, S., Yambélé, A., Gillikin, D. P., Teodoru, C., Darchambeau, F., Lambert, T., and Borges, A. V.: Contrasting biogeochemical characteristics of the Oubangui River and tributaries (Congo River basin), Sci. Rep.-UK, 4, 5402, https://doi.org/10.1038/srep05402, 2015.
Bowden, W. B.: Arctic LTER streams chemistry Toolik Field Station, Alaska 1978 to 2019., Environmental Data Initiative, https://doi.org/10.6073/pasta/3faacd18b63b3bacc5a0dbd6f09660e1, 2021.
Bower, S.: Effects of storms on nitrate removal and greenhouse gas emissions from fluvial wetland dominated surface water flow paths, MS thesis, University of New Hampshire, Durham, NH, USA, https://scholars.unh.edu/thesis/1376/ (last access: 12 June 2023), 2020.
Bresney, S. R., Moseman-Valtierra, S., and Snyder, N. P.: Observations of greenhouse gases and nitrate concentrations in a Maine river and fringing wetland, Northeast. Nat., 22, 120–143, https://doi.org/10.1656/045.022.0125, 2015.
Bretz, K. A., Jackson, A. R., Rahman, S., Monroe, J. M., and Hotchkiss, E. R.: Integrating ecosystem patch contributions to stream corridor carbon dioxide and methane fluxes, J. Geophys. Res. Biogeo., 126, e2021JG006313, https://doi.org/10.1029/2021JG006313, 2021a.
Bretz, K. A., Jackson, A. R., Rahman, S., Monroe, J. M., and Hotchkiss, E. R.: Integrating EcosystemPatchContributionsStreamCO2CH4_Data, HydroShare, https://doi.org/10.4211/hs.ab2b33f27b3b4a0ca9a8ce4b8936753f, 2021b.
Brown, R. S. and Hershey, A. E.: Potential effects of the invasive bivalve Corbicula fluminea on methane cycling processes in an urban stream, Biogeochemistry, 144, 181–195, https://doi.org/10.1007/s10533-019-00578-1, 2019.
Bugna, G. C., Chanton, J. P., Cable, J. E., Burnett, W. C., and Cable, P. H.: The importance of groundwater discharge to the methane budgets of nearshore and continental shelf waters of the northeastern Gulf of Mexico, Geochim. Cosmochim. Ac., 60, 4735–4746, https://doi.org/10.1016/S0016-7037(96)00290-6, 1996.
Buriánková, I., Brablcová, L., Mach, V., Hýblová, A., Badurová, P., Cupalová, J., Čáp, L., and Rulík, M.: Methanogens and methanotrophs distribution in the hyporheic sediments of a small lowland stream, Fundam. Appl. Limnol., 181, 87–102, https://doi.org/10.1127/1863-9135/2012/0283, 2012.
Burns, R., Wynn, P. M., Barker, P., McNamara, N., Oakley, S., Ostle, N., Stott, A. W., Tuffen, H., Zhou, Z., Tweed, F. S., Chesler, A., and Stuart, M.: Direct isotopic evidence of biogenic methane production and efflux from beneath a temperate glacier, Sci. Rep.-UK, 8, 17118, https://doi.org/10.1038/s41598-018-35253-2, 2018.
Burrows, R. M., van de Kamp, J., Bodrossy, L., Venarsky, M., Coates-Marnane, J., Rees, G., Jumppanen, P., and Kennard, M. J.: Methanotroph community structure and processes in an inland river affected by natural gas macro-seeps, FEMS Microbiol. Ecol., 97, fiab130, https://doi.org/10.1093/femsec/fiab130, 2021.
Bussmann, I.: Distribution of methane in the Lena Delta and Buor-Khaya Bay, Russia, Biogeosciences, 10, 4641–4652, https://doi.org/10.5194/bg-10-4641-2013, 2013a.
Bussmann, I.: Methane concentration and isotopic composition (δ13C) in the waters of the Lena River and the Laptev Sea, in the years 2008, 2009 and 2010, PANGAEA, https://doi.org/10.1594/PANGAEA.817302, 2013b.
Bussmann, I. and Fedorova, I. V.: Dissolved methane concentrations under ice cover in the Lena Delta area, PANGAEA, https://doi.org/10.1594/PANGAEA.905776, 2019.
Bussmann, I., Fedorova, I. V., Juhls, B., Overduin, P. P., and Winkel, M.: Dissolved methane concentrations and oxidation rates in the Lena Delta area, 2016–2018, 2 datasets, PANGAEA, https://doi.org/10.1594/PANGAEA.920015, 2020.
Bussmann, I., Fedorova, I., Juhls, B., Overduin, P. P., and Winkel, M.: Methane dynamics in three different Siberian water bodies under winter and summer conditions, Biogeosciences, 18, 2047–2061, https://doi.org/10.5194/bg-18-2047-2021, 2021.
Call, M., Sanders, C. J., Enrich-Prast, A., Sanders, L., Marotta, H., Santos, I. R., and Maher, D. T.: Metadata: Radon-traced pore-water as a potential source of CO2 and CH4 to receding black and clear water environments in the Amazon Basin: Black and clear water environments in the Amazon Basin, figshare, https://figshare.com/s/95945208c33e8800f02c (last access: 12 June 2023), 2018a.
Call, M., Sanders, C. J., Enrich-Prast, A., Sanders, L., Marotta, H., Santos, I. R., and Maher, D. T.: Radon-traced pore-water as a potential source of CO2 and CH4 to receding black and clear water environments in the Amazon Basin: Black and clear water environments in the Amazon Basin, Limnol. Oceanogr. Lett., 3, 375–383, https://doi.org/10.1002/lol2.10089, 2018b.
Campeau, A.: Dataset for manuscript: Stable carbon isotopes reveal soil-stream DIC linkages in contrasting headwater catchments (Version 1.0), Uppsala University Publications, http://urn.kb.se/resolve?urn=urn:nbn:se:uu:diva-335488 (last access: 12 June 2023), 2018.
Campeau, A., Lapierre, J.-F., Vachon, D., and del Giorgio, P. A.: Regional contribution of CO2 and CH4 fluxes from the fluvial network in a lowland boreal landscape of Québec: CO2 and CH4 emission from boreal rivers, Global Biogeochem. Cy., 28, 57–69, https://doi.org/10.1002/2013GB004685, 2014.
Campeau, A., Bishop, K. H., Billett, M. F., Garnett, M. H., Laudon, H., Leach, J. A., Nilsson, M. B., Öquist, M. G., and Wallin, M. B.: Aquatic export of young dissolved and gaseous carbon from a pristine boreal fen: Implications for peat carbon stock stability, Glob. Change Biol., 23, 5523–5536, https://doi.org/10.1111/gcb.13815, 2017.
Campeau, A., Bishop, K., Nilsson, M. B., Klemedtsson, L., Laudon, H., Leith, F. I., Öquist, M., and Wallin, M. B.: Stable carbon isotopes reveal soil-stream DIC linkages in contrasting headwater catchments, J. Geophys. Res.-Biogeo., 123, 149–167, https://doi.org/10.1002/2017JG004083, 2018.
Carey, C. C., Woelmer, W. M., Maze, J. T., and Hounshell, A. G.: Manually-collected discharge data for multiple inflow tributaries entering Falling Creek Reservoir and Beaverdam Reservoir, Vinton, Virginia, USA in 2019, Environmental Data Initiative, https://doi.org/10.6073/pasta/4d8e7b7bedbc6507b307ba2d5f2cf9a2, 2019.
Carey, C. C., Hounshell, A. G., Lofton, M. E., Birgand, F., Bookout, B. J., Corrigan, R. S., Gerling, A. B., McClure, R. P., and Woelmer, W. M.: Discharge time series for the primary inflow tributary entering Falling Creek Reservoir, Vinton, Virginia, USA 2013-2021, Environmental Data Initiative, https://doi.org/10.6073/pasta/8d22a432aac5560b0f45aa1b21ae4746, 2021a.
Carey, C. C., Wander, H. L., Woelmer, W. M., Lofton, M. E., Breef-Pilz, A., Doubek, J. P., Gerling, A. B., Hounshell, A. G., McClure, R. P., and Niederlehner, B. R.: Water chemistry time series for Beaverdam Reservoir, Carvins Cove Reservoir, Falling Creek Reservoir, Gatewood Reservoir, and Spring Hollow Reservoir in southwestern Virginia, USA 2013–2020, Environmental Data Initiative, https://doi.org/10.6073/pasta/8d83ef7ec202eca9192e3da6dd34a4e0, 2021b.
Carey, C. C., Hounshell, A. G., McClure, R. P., Gerling, A. B., Lewis, A. S. L., and Niederlehner, B. R.: Time series of dissolved methane and carbon dioxide concentrations for Falling Creek Reservoir and Beaverdam Reservoir in southwestern Virginia, USA during 2015–2021, Environmental Data Initiative, https://doi.org/10.6073/pasta/2fb836492aace4c13b7962f2718be8e5, 2022.
Carpenter, C., Wickland, K. P., Dornblaser, M. M., Clow, D. W., Koch, J. C., and Dantoin, E. D.: Wetland stream water quality data for West Twin Creek, AK, Allequash Creek, WI, and Big Thompson River, CO, 2010–2020: U.S. Geological Survey data release, https://doi.org/10.5066/P9WYS23U, 2021.
Castro-Morales, K., Canning, A., Körtzinger, A., Göckede, M., Küsel, K., Overholt, W.A., Wichard, T., Redlich, S., Arzberger, S., Kolle, O., and Zimov, N.: Data published in manuscript “Effects of reversal of water flow in an Arctic floodplain river on fluvial emissions of CO2 and CH4” by Castro-Morales et al., Zenodo, https://doi.org/10.5281/zenodo.5758728, 2021.
Castro-Morales, K., Canning, A., Körtzinger, A., Göckede, M., Küsel, K., Overholt, W. A., Wichard, T., Redlich, S., Arzberger, S., Kolle, O., and Zimov, N.: Effects of reversal of water flow in an Arctic floodplain river on fluvial emissions of CO2 and CH4, J. Geophys. Res.-Biogeo., 127, e2021JG006485, https://doi.org/10.1029/2021jg006485, 2022.
Chamberlain, S. D., Boughton, E. H., and Sparks, J. P.: Underlying ecosystem emissions exceed cattle-emitted methane from subtropical lowland pastures, Ecosystems, 18, 933–945, https://doi.org/10.1007/s10021-015-9873-x, 2015.
Chan, C.-N., Shi, H., Liu, B., and Ran, L.: CO2 and CH4 emissions from an arid fluvial network on the Chinese Loess Plateau, Water, 13, 1614, https://doi.org/10.3390/w13121614, 2021.
Chen, C.-T. A., Wang, S.-L., Lu, X.-X., Zhang, S.-R., Lui, H.-K., Tseng, H.-C., Wang, B.-J., and Huang, H.-I.: Hydrogeochemistry and greenhouse gases of the Pearl River, its estuary and beyond, Quatern. Int., 186, 79–90, https://doi.org/10.1016/j.quaint.2007.08.024, 2008.
Chen, S., Wang, D., Ding, Y., Yu, Z., Liu, L., Li, Y., Yang, D., Gao, Y., Tian, H., Cai, R., and Chen, Z.: Ebullition controls on CH4 emissions in an urban, eutrophic river: A potential time-scale bias in determining the aquatic CH4 flux, Environ. Sci. Technol., 55, 7287–7298, https://doi.org/10.1021/acs.est.1c00114, 2021.
Clarizia, P. E.: Seasonal methane and carbon dioxide emissions along a temperate fluvial wetland dominated river continuum, MS thesis, University of New Hampshire, Durham, NH, USA, 40 pp., https://scholars.unh.edu/thesis/1322/ (last access: 12 June 2023), 2019.
Clayer, F., Thrane, J.-E., Brandt, U., Dörsch, P., and Wit, H. A.: Boreal headwater catchment as hot spot of carbon processing from headwater to fjord, J. Geophys. Res.-Biogeo., 126, e2021JG006359, https://doi.org/10.1029/2021JG006359, 2021a.
Clayer, F., Thrane, J. -E., Brandt, U., Dörsch, P., and de Wit, H.: Dataset for “Boreal headwater catchment as hot spot of carbon processing from headwater to fjord” Clayer et al., HydroShare, https://doi.org/10.4211/hs.b143432cfe72462fac90376631c9a2b3, 2021b.
Clilverd, H. M., Jones, J. B., and Kielland, K.: Nitrogen retention in the hyporheic zone of a glacial river in interior Alaska, Biogeochemistry, 88, 31–46, https://doi.org/10.1007/s10533-008-9192-9, 2008.
Comer-Warner, S., Krause, S., Gooddy, D. C., Ullah, S., and Wexler, S. K.: Seasonal streambed carbon and nitrogen cycling (including greenhouse gases) in an agriculturally-impacted stream. Measured at Wood Brook UK, 2016–2017, NERC Environmental Information Data Centre, https://doi.org/10.5285/00601260-285e-4ffa-b381-340b51a7ec50, 2018.
Comer-Warner, S. A., Gooddy, D. C., Ullah, S., Glover, L., Percival, A., Kettridge, N., and Krause, S.: Seasonal variability of sediment controls of carbon cycling in an agricultural stream, Sci. Total Environ., 688, 732–741, https://doi.org/10.1016/j.scitotenv.2019.06.317, 2019.
Cotovicz, L. C., Ribeiro, R. P., Régis, C. R., Bernardes, M., Sobrinho, R., Vidal, L. O., Tremmel, D., Knoppers, B. A., and Abril, G.: Greenhouse gas emissions (CO2 and CH4) and inorganic carbon behavior in an urban highly polluted tropical coastal lagoon (SE, Brazil), Environ. Sci. Pollut. R., 28, 38173–38192, https://doi.org/10.1007/s11356-021-13362-2, 2021.
Cowley, K., Looman, A., Maher, D. T., and Fryirs, K.: Geomorphic controls on fluvial carbon exports and emissions from upland swamps in eastern Australia, Sci. Total Environ., 618, 765–776, https://doi.org/10.1016/j.scitotenv.2017.08.133, 2018.
Crawford, J. and Stanley, E.: Greenhouse gas emissions from streams at North Temperate Lakes LTER 2012, Environmental Data Initiative, https://doi.org/10.6073/pasta/000c46ce5c2ded2cefbddb596fca3ce2, 2020.
Crawford, J. T.: Carbon gas emissions from headwater streams, Univesity of Wisconsin-Madison, Madison, WI, USA, unpublished, 2014.
Crawford, J., Stanley, E., and Loken, L.: Ebullitive methane emissions from oxygenated wetland streams at North Temperate Lakes LTER 2013, Environmental Data Initiative, https://doi.org/10.6073/pasta/7842765e6520b62af6fd4d95c838f177, 2020.
Crawford, J. T. and Stanley, E. H.: Controls on methane concentrations and fluxes in streams draining human-dominated landscapes, Ecol. Appl., 26, 1581–1591, https://doi.org/10.1890/15-1330, 2016.
Crawford, J. T., Striegl, R. G., Wickland, K. P., Dornblaser, M. M., and Stanley, E. H.: Emissions of carbon dioxide and methane from a headwater stream network of interior Alaska, J. Geophys. Res.-Biogeo., 118, 482–494, https://doi.org/10.1002/jgrg.20034, 2013.
Crawford, J. T., Lottig, N. R., Stanley, E. H., Walker, J. F., Hanson, P. C., Finlay, J. C., and Striegl, R. G.: CO2 and CH4 emissions from streams in a lake-rich landscape: Patterns, controls, and regional significance, Global Biogeochem. Cy., 28, 197–210, https://doi.org/10.1002/2013GB004661, 2014a.
Crawford, J. T., Stanley, E. H., Spawn, S. A., Finlay, J. C., Loken, L. C., and Striegl, R. G.: Ebullitive methane emissions from oxygenated wetland streams, Glob. Change Biol., 20, 3408–3422, https://doi.org/10.1111/gcb.12614, 2014b.
Crawford, J. T., Dornblaser, M. M., Stanley, E. H., Clow, D. W., and Striegl, R. G.: Source limitation of carbon gas emissions in high-elevation mountain streams and lakes, J. Geophys. Res.-Biogeo., 120, 952–964, https://doi.org/10.1002/2014JG002861, 2015.
Crawford, J. T., Loken, L. C., Stanley, E. H., Stets, E. G., Dornblaser, M. M., and Striegl, R. G.: Basin scale controls on CO2 and CH4 emissions from the Upper Mississippi River: Mississippi River Greenhouse Gases, Geophys. Res. Lett., 43, 1973–1979, https://doi.org/10.1002/2015GL067599, 2016.
Dahm, C. N., Carr, D. L., and Coleman, R. L.: Anaerobic carbon cycling in stream ecosystems, SIL Proceedings 1922–2010, 24, 1600–1604, https://doi.org/10.1080/03680770.1989.11899028, 1991.
Dawson, J. J. C., Billett, M. F., Hope, D., Palmer, S. M., and Deacon, C. M.: Sources and sinks of aquatic carbon in a peatland stream continuum, Biogeochemistry, 70, 71–92, https://doi.org/10.1023/B:BIOG.0000049337.66150.f1, 2004.
de Angelis, M. A. and Lilley, M. D.: Methane in surface waters of Oregon estuaries and rivers, Limnol. Oceanogr., 32, 716–722, https://doi.org/10.4319/lo.1987.32.3.0716, 1987.
de Angelis, M. A. and Scranton, M. I.: Fate of methane in the Hudson River and Estuary, Global Biogeochem. Cy., 7, 509–523, https://doi.org/10.1029/93GB01636, 1993.
Dean, J. F., Billett, M. F., Baxter, R., Dinsmore, K. J., Lessels, J. S., Street, L. E., Subke, J.-A., Tetzlaff, D., Washbourne, I., and Wookey, P. A.: Biogeochemistry of “pristine” freshwater stream and lake systems in the western Canadian Arctic, Biogeochemistry, 130, 191–213, https://doi.org/10.1007/s10533-016-0252-2, 2016.
Dean, J. F., Meisel, O. H., Martyn Rosco, M., Marchesini, L. B., Garnett, M. H., Lenderink, H., van Logtestijn, R., Borges, A. V., Bouillon, S., Lambert, T., Röckmann, T., Maximov, T., Petrov, R., Karsanaev, S., Aerts, R., van Huissteden, J., Vonk, J. E., and Dolman, A. J.: East Siberian Arctic inland waters emit mostly contemporary carbon, Nat. Commun, 11, 1627, https://doi.org/10.1038/s41467-020-15511-6, 2020.
Deirmendjian, L., Anschutz, P., Morel, C., Mollier, A., Augusto, L., Loustau, D., Cotovicz, L. C., Buquet, D., Lajaunie, K., Chaillou, G., Voltz, B., Charbonnier, C., Poirier, D., and Abril, G.: Importance of the vegetation-groundwater-stream continuum to understand transformation of biogenic carbon in aquatic systems – A case study based on a pine-maize comparison in a lowland sandy watershed (Landes de Gascogne, SW France), Sci. Total Environ., 661, 613–629, https://doi.org/10.1016/j.scitotenv.2019.01.152, 2019.
DelSontro, T., Perez, K. K., Sollberger, S., and Wehrli, B.: Methane dynamics downstream of a temperate run-of-the-river reservoir: CH4 dynamics below a run-of-the-river reservoir, Limnol. Oceanogr., 61, S188–S203, https://doi.org/10.1002/lno.10387, 2016.
Deng, O., Li, X., Deng, L., Zhang, S., Gao, X., Lan, T., Zhou, W., Tian, D., Xiao, Y., Yang, J., Ou, D., and Luo, L.: Emission of CO2 and CH4 from a multi-ditches system in rice cultivation region: Flux, temporal-spatial variation and effect factors, J. Environ. Manage., 270, 110918, https://doi.org/10.1016/j.jenvman.2020.110918, 2020.
Descloux, S., Chanudet, V., Serça, D., and Guérin, F.: Methane and nitrous oxide annual emissions from an old eutrophic temperate reservoir, Sci. Total Environ., 598, 959–972, https://doi.org/10.1016/j.scitotenv.2017.04.066, 2017.
Deshmukh, C., Guérin, F., Labat, D., Pighini, S., Vongkhamsao, A., Guédant, P., Rode, W., Godon, A., Chanudet, V., Descloux, S., and Serça, D.: Low methane (CH4) emissions downstream of a monomictic subtropical hydroelectric reservoir (Nam Theun 2, Lao PDR), Biogeosciences, 13, 1919–1932, https://doi.org/10.5194/bg-13-1919-2016, 2016.
Dinsmore, K. J., Billett, M. F., Skiba, U. M., Rees, R. M., Drewer, J., and Helfter, C.: Role of the aquatic pathway in the carbon and greenhouse gas budgets of a peatland catchment, Glob. Change Biol., 16, 2750–2762, https://doi.org/10.1111/j.1365-2486.2009.02119.x, 2010.
Dinsmore, K. J., Billett, M. F., Dyson, K. E., Harvey, F., Thomson, A. M., Piirainen, S., and Kortelainen, P.: Stream water hydrochemistry as an indicator of carbon flow paths in Finnish peatland catchments during a spring snowmelt event, Sci. Total Environ., 409, 4858–4867, https://doi.org/10.1016/j.scitotenv.2011.07.063, 2011.
Dinsmore, K. J., Billett, M. F., and Dyson, K. E.: Five year record of aquatic carbon and greenhouse gas concentrations from Auchencorth Moss, NERC Environmental Information Data Centre, https://doi.org/10.5285/3f0820a7-a8c8-4dd7-a058-8db79ba9c7fe, 2013a.
Dinsmore, K. J., Billett, M. F., and Dyson, K. E.: Temperature and precipitation drive temporal variability in aquatic carbon and GHG concentrations and fluxes in a peatland catchment, Glob. Change Biol., 19, 2133–2148, https://doi.org/10.1111/gcb.12209, 2013b.
Dinsmore, K. J., Murphey, O., Leith, F., and Carfrae, J.: Aquatic carbon and greenhouse gas concentrations in the Auchencorth Moss catchment following drain blocking, NERC Environmental Information Data Centre, https://doi.org/10.5285/88ffbf44-0ec0-41d6-9814-04bc3535cd84, 2016.
Dodd, A.: Flow regime influences on stream and riparian soil carbon dynamics in the Ozark Highlands and Boston Mountains of Arkansas, PhD, University of Arkansas, Fayetteville, AR, USA, 143 pp., https://scholarworks.uark.edu/etd/2911/ (last access: 12 June 2023), 2018.
Dornblaser, M. M. and Halm, D. R. (Eds.): Water and sediment quality of the Yukon River and its tributaries, from Eagle to St. Marys, Alaska, 2002–2003, U.S. Geological Survey Open-File Report 2006-1228, Reston, VA, 202 pp., https://doi.org/10.3133/ofr20061228, 2006.
Druschke, C. G.: Geomorphic and ecological effects of restoration and flood events on stream in the Driftless Area of Wisconsin, MS thesis, University of Wisconsin-Madison, Madison, WI, USA, 59 pp., https://search.library.wisc.edu/catalog/9913456953802121 (last access: 12 June 2023), 2021.
Druschke, C. G., Booth, E. G., and Stanley, E. H.: Stream restoration and flood impacts in the Kickapoo River Watershed, Wisconsin, 2019, Environmental Data Initiative, https://doi.org/10.6073/pasta/4905369228d80920974f555a2dd12229, 2022.
Dyson, K. E., Billett, M. F., Dinsmore, K. J., Harvey, F., Thomson, A. M., Piirainen, S., and Kortelainen, P.: Release of aquatic carbon from two peatland catchments in E. Finland during the spring snowmelt period, Biogeochemistry, 103, 125–142, https://doi.org/10.1007/s10533-010-9452-3, 2011.
Einarsdottir, K., Wallin, M. B., and Sobek, S.: High terrestrial carbon load via groundwater to a boreal lake dominated by surface water inflow: Carbon input to a lake via Groundwater, J. Geophys. Res.-Biogeo., 122, 15–29, https://doi.org/10.1002/2016JG003495, 2017.
Emmerton, C. A., St. Louis, V. L., Lehnherr, I., Humphreys, E. R., Rydz, E., and Kosolofski, H. R.: The net exchange of methane with high Arctic landscapes during the summer growing season, Biogeosciences, 11, 3095–3106, https://doi.org/10.5194/bg-11-3095-2014, 2014.
Evans, C. D., Peacock, M., Green, S. M., Holden, J., Chapman, P. J., Lebron, I., Callaghan, N., Grayson, R., and Baird, A. J.: The impact of ditch blocking on fluvial carbon export from a UK blanket bog, Hydrol. Process., 32, 2141–2154, https://doi.org/10.1002/hyp.13158, 2018.
Federov, Y. A., Khoroshevskaya, V. O., and Tambieva, N. S.: Variations in methane concentrations in the water of the Don River and Taganrog Bay under the effect of natural factors, Water Resour., 30, 89–93, https://doi.org/10.1023/A:1022059903167, 2003.
Flanagan, L. B., Nikkel, D. J., Scherloski, L. M., Tkach, R. E., Smits, K. M., Selinger, L. B., and Rood, S. B.: Multiple processes contribute to methane emission in a riparian cottonwood forest ecosystem, New Phytol., 229, 1970–1982, https://doi.org/10.1111/nph.16977, 2020.
Flessa, H., Rodionov, A., Guggenberger, G., Fuchs, H., Magdon, P., Shibistova, O., Zrazhevskaya, G., Mikheyeva, N., Kasansky, O. A., and Blodau, C.: Landscape controls of CH4 fluxes in a catchment of the forest tundra ecotone in northern Siberia, Glob. Change Biol.,, 14, 2040–2056, https://doi.org/10.1111/j.1365-2486.2008.01633.x, 2008.
Flury, S. and Ulseth, A. J.: Exploring the sources of unexpected high methane concentrations and fluxes from Alpine headwater streams, Geophys. Res. Lett., 46, 6614–6625, https://doi.org/10.1029/2019GL082428, 2019.
Foks, S. S., Stackpoole, S. M., Dornblaser, M. M., Whiddon, E. T., Breitmeyer, S. E., Campbell, D. A., Crawford, J. T., Metcalf, E. B., Stets, E. G., Uhle, B. A., Voss, B. M., Wickland, K. P., and Striegl, R. G.: Water quality, quantity, and gas fluxes of the Upper Mississippi River basin (WY 2012-2016), U.S. Geological Survey data release, https://doi.org/10.5066/F7P849S4, 2018.
Foks, S. S., Dornblaser, M. M., Butman, D. E., Campbell, D. A., Koch, J. C., Li, Z., Whiddon, E. T., Wickland, K. P., Striegl, R., Bogard, M. J., Spencer, R. G. M., Textor, S. R., and Johnston, S. E.: Water quality and gas fluxes of Interior Alaska (2014-2018), U.S. Geological Survey data release, https://doi.org/10.5066/P9C6BDBQ, 2020.
Ford, T. E. and Naiman, R. J.: Alteration of carbon cycling by beaver: methane evasion rates from boreal forest streams and rivers, Can. J. Zool., 66, 529–533, https://doi.org/10.1139/z88-076, 1988.
Galantini, L., Lapierre, J.-F., and Maranger, R.: How are greenhouse gases coupled across seasons in a large temperate river with differential land use?, Ecosystems, 24, 2007–2027, https://doi.org/10.1007/s10021-021-00629-5, 2021.
Gareis, J. A. L. and Lesack, L. F. W.: Ice-out and freshet fluxes of CO2 and CH4 across the air–water interface of the channel network of a great Arctic delta, the Mackenzie, Polar Res., 39, 3528, https://doi.org/10.33265/polar.v39.3528, 2020.
Gar'kusha, D. N. and Fedorov, Y. A.: Methane in the water and bottom sediments of the mouth area of the Severnaya Dvina River during the winter time, Oceanology, 54, 160–169, https://doi.org/10.1134/S000143701402009X, 2014.
Gar'kusha, D. N. and Fedorov, Y. A.: Distribution of methane concentration in coastal areas of the Gulf of Petrozavodsk, Lake Onega, Water Resour., 42, 331–339, https://doi.org/10.1134/S0097807815030045, 2015.
Gar'kusha, D. N., Fedorov, Y. A., and Khromov, M. I.: Methane in the water and bottom sediments of the mouth area of the Severnaya Dvina River (White Sea), Oceanology, 50, 498–512, https://doi.org/10.1134/S0001437010040065, 2010a.
Gar'kusha, D. N., Fedorov, Y., and Khromov, M. I.: Methane in waters and bottom sediments of the North (Severnaya) Dvina mouth area in 2004–2006, PANGAEA, https://doi.org/10.1594/PANGAEA.763838, 2010b.
Garnier, J., Vilain, G., Silvestre, M., Billen, G., Jehanno, S., Poirier, D., Martinez, A., Decuq, C., Cellier, P., and Abril, G.: Budget of methane emissions from soils, livestock and the river network at the regional scale of the Seine basin (France), Biogeochemistry, 116, 199–214, https://doi.org/10.1007/s10533-013-9845-1, 2013.
Gatland, J. R., Santos, I. R., Maher, D. T., Duncan, T. M., and Erler, D. V.: Carbon dioxide and methane emissions from an artificially drained coastal wetland during a flood: Implications for wetland global warming potential, J. Geophys. Res.-Biogeo., 119, 1698–1716, https://doi.org/10.1002/2013JG002544, 2014.
Gatti, R. C., Callaghan, T. V., Rozhkova-Timina, I., Dudko, A., Lim, A., Vorobyev, S. N., Kirpotin, S. N., and Pokrovsky, O. S.: The role of Eurasian beaver (Castor fiber) in the storage, emission and deposition of carbon in lakes and rivers of the River Ob flood plain, western Siberia, Sci. Total Environ., 644, 1371–1379, https://doi.org/10.1016/j.scitotenv.2018.07.042, 2018.
Gómez-Gener, L., Obrador, B., von Schiller, D., Marcé, R., Casas-Ruiz, J. P., Proia, L., Acuña, V., Catalán, N., Muñoz, I., and Koschorreck, M.: Hot spots for carbon emissions from Mediterranean fluvial networks during summer drought, Biogeochemistry, 125, 409–426, https://doi.org/10.1007/s10533-015-0139-7, 2015.
Gómez-Gener, L., Gubau, M., von Schiller, D., Marcé, R., and Obrador, B.: Effect of small water retention structures on diffusive CO2 and CH4 emissions along a highly impounded river, Inland Waters, 8, 449–460, https://doi.org/10.1080/20442041.2018.1457846, 2018.
Gondwe, M. J. and Masamba, W. R. L.: Spatial and temporal dynamics of diffusive methane emissions in the Okavango Delta, northern Botswana, Africa, Wetl. Ecol. Manag., 22, 63–78, https://doi.org/10.1007/s11273-013-9323-5, 2014.
Gong, X. J., Wang, X. F., Yuan, X. Z., Liu, T. T., and Hou, C. L.: Effects of field town developments on the dissolved and diffusion fluxes of greenhouse gasses in Heishuitan River basin, Chongqing, Acta Ecol. Sin., 39, 8425–8441, https://www.ecologica.cn/html/2019/22/stxb201810112205.htm (last access: 12 June 2023), 2019.
Gonzalez-Valencia, R. Sepulveda-Jauregui, A., Martinez-Cruz, K. Hoyos-Santillan, J. Dendooven, L., and Thalasso, L.: Methane emissions from Mexican freshwater bodies: correlations with water pollution, Hydrobiologia, 721, 9–22, https://doi.org/10.1007/s10750-013-1632-4, 2014.
Grieve, P. L., Hynek, S. A., Heilweil, V., Sowers, T., Llewellyn, G., Yoxtheimer, D., Solomon, D. K., and Brantley, S. L.: Using environmental tracers and modelling to identify natural and gas well-induced emissions of methane into streams, Appl. Geochem., 91, 107–121, https://doi.org/10.1016/j.apgeochem.2017.12.022, 2018.
Gu, C., Waldron, S., and Bass, A. M.: Carbon dioxide, methane, and dissolved carbon dynamics in an urbanized river system, Hydrol. Process., 35, e14360, https://doi.org/10.1002/hyp.14360, 2021.
Guo, Y., Song, C., Wang, L., Tan, W., Wang, X., Cui, Q., and Wan, Z.: Concentrations, sources, and export of dissolved CH4 and CO2 in rivers of the permafrost wetlands, northeast China, Ecol. Eng., 90, 491–497, https://doi.org/10.1016/j.ecoleng.2015.10.004, 2016.
Halm, D. R. and Dornblaser, M. M. (Eds.): Water and sediment quality in the Yukon River and its tributaries, between Atlin, British Columbia, Canada, and Eagle, Alaska, 2004, U.S. Geological Survey Open-File Report 2007–1197, Reston, VA, 120 pp., https://doi.org/10.3133/ofr20071197, 2007.
Hamilton, S. K., Sippel, S., and Melack, J. M.: Oxygen depletion and carbon dioxide and methane production in waters of the Pantanal wetland of Brazil, Biogeochemistry, 30, 115–141, https://doi.org/10.1007/bf00002727, 1995.
Han, Y.: Greenhouse gases emission characteristics of rivers in Nanjing and the influencing factors, MS thesis, Nanjing University of Information Science and Technology, Nanjing, China, https://kns.cnki.net/kcms/detail/detail.aspx?dbname=cmfd201401&filename=1013340872.nh (last access: 12 June 2023), 2013.
Han, Y., Zhang, G., and Zhao, Y.: Distribution and fluxes of methane in tropical rivers and lagoons of eastern Hainan, J. Trop. Oceanogr., 31, 87–95, http://www.jto.ac.cn/CN/10.11978/j.issn.1009-5470.2012.02.012, 2012.
Hao, X., Ruihong, Y., Zhuangzhuang, Z., Zhen, Q., Xixi, L., Tingxi, L., and Ruizhong, G.: Greenhouse gas emissions from the water–air interface of a grassland river: a case study of the Xilin River, Sci. Rep.-UK, 11, 2659, https://doi.org/10.1038/s41598-021-81658-x, 2021.
Harms, T. K. and Godsey, S. E.: Collaborative Research: Climate-mediated coupling of hydrology and biogeochemistry in arctic hillslopes, Arctic Data Center, https://doi.org/10.18739/a23r0ps5p, 2018.
Harms, T. K., Rocher-Ros, G., and Godsey, S. E.: Emission of greenhouse gases from water tracks draining Arctic hillslopes, J. Geophys. Res.-Biogeo., 125, e2020JG005889, https://doi.org/10.1029/2020JG005889, 2020.
Harrison, J. A., Matson, P. A., and Fendorf, S. E.: Effects of a diel oxygen cycle on nitrogen transformations and greenhouse gas emissions in a eutrophied subtropical stream, Aquat. Sci., 67, 308–315, https://doi.org/10.1007/s00027-005-0776-3, 2005.
Hattenberger, D. M.: Comparing carbon dioxide and methane emissions from restored and unrestored sections of three North Carolina streams, MS thesis, North Carolina State University, Raliegh, NC, USA, 103 pp., http://www.lib.ncsu.edu/resolver/1840.20/35725 (last access: 14 June 2023), 2018.
Hayakawa, A., Ikeda, S., Tsushima, R., Ishikawa, Y., and Hidaka, S.: Spatial and temporal variations in nutrients in water and riverbed sediments at the mouths of rivers that enter Lake Hachiro, a shallow eutrophic lake in Japan, Catena, 133, 486–494, https://doi.org/10.1016/j.catena.2015.04.009, 2015.
He, B., He, J., Wang, J., Li, J., and Wang, F.: Characteristics of GHG flux from water–air interface along a reclaimed water intake area of the Chaobai River in Shunyi, Beijing, Atmos. Environ., 172, 102–108, https://doi.org/10.1016/j.atmosenv.2017.10.060, 2018.
Heilweil, V. M., Stolp, B. J., Kimball, B. A., Susong, D. D., Marston, T. M., and Gardner, P. M.: A stream-based methane monitoring approach for evaluating groundwater impacts associated with unconventional gas development, Groundwater, 51, 511–524, https://doi.org/10.1111/gwat.12079, 2013.
Heilweil, V. M., Risser, D. W., Conger, R. W., Grieve, P. L., and Hyneck, S. A.: Estimation of methane concentrations and loads in groundwater discharge to Sugar Run, Lycoming County, Pennsylvania, U.S. Geological Survey Open File Report 2014–1126., Reston, VA, 31 p., https://doi.org/10.3133/ofr20141126, 2014.
Heilweil, V. M., Solomon, D. K., Darrah, T. H., Gilmore, T. E., and Genereux, D. P.: Gas-tracer experiment for evaluating the fate of methane in a coastal plain stream: Degassing versus in-stream oxidation, Environ. Sci. Technol., 50, 10504–10511, https://doi.org/10.1021/acs.est.6b02224, 2016.
Hendriks, D. M. D., van Huissteden, J., and Dolman, A. J.: Multi-technique assessment of spatial and temporal variability of methane fluxes in a peat meadow, Agr. Forest Meteorol., 150, 757–774, https://doi.org/10.1016/j.agrformet.2009.06.017, 2010.
Heppell, C. M. and Binley, A.: Hampshire Avon: Daily discharge, stage and water chemistry data from four tributaries (Sem, Nadder, West Avon, Ebble), NERC Environmental Information Data Centre, https://doi.org/10.5285/0dd10858-7b96-41f1-8db5-e7b4c4168af5, 2016a.
Heppell, C. M. and Binley, A.: Hampshire Avon: Vertical head gradient, saturated hydraulic conductivity and pore water chemistry data from six river reaches, NERC Environmental Information Data Centre, https://doi.org/10.5285/d82a04ce-f04d-40b4-9750-1a2bf7dc29a3, 2016b.
Herman-Mercer, N. M.: Water-quality data from the Yukon River Basin in Alaska and Canada, U.S. Geological Survey data release, https://doi.org/10.5066/f77d2s7b, 2016.
Herreid, A. M., Wymore, A. S., Varner, R. K., Potter, J. D., and McDowell, W. H.: Divergent controls on stream greenhouse gas concentrations across a land-use gradient, Ecosystems, 24, 1299–1316, https://doi.org/10.1007/s10021-020-00584-7, 2020a.
Herreid, A. M., Wymore, A. S., Varner, R. K., Potter, J. D., and McDowell, W. H.: Divergent controls on stream greenhouse gas concentrations across a land use gradient, HydroShare, https://doi.org/10.4211/hs.2679ce1a6d514b30a54459893557dfe7, 2020b.
Herrero Ortega, S., Romero González-Quijano, C., Casper, P., Singer, G. A., and Gessner, M. O.: Methane emissions from contrasting urban freshwaters: Rates, drivers, and a whole-city footprint, Glob. Change Biol., 25, 4234–4243, https://doi.org/10.1111/gcb.14799, 2019.
Hlaváčová, E., Rulík, M., and Čáp, L.: Anaerobic microbial metabolism in hyporheic sediment of a gravel bar in a small lowland stream, River Res. Appl., 21, 1003–1011, https://doi.org/10.1002/rra.866, 2005.
Hlaváčová, E., Rulík, M., Čáp, L., and Mach, V.: Greenhouse gas (CO2, CH4, N2O) emissions to the atmosphere from a small lowland stream in Czech Republic, Arch. Hydrobiol., 165, 339–353, https://doi.org/10.1127/0003-9136/2006/0165-0339, 2006.
Ho, L., Jerves-Cobo, R., Barthel, M., Six, J., Bode, S., Boeckx, P., and Goethals, P.: Dissolved gas concentrations in Cuenca river systems (Ecuador), Environmental Data Initiative, https://doi.org/10.6073/pasta/545502bf79b3e4e03bad2c5816375b01, 2021.
Ho, L., Jerves-Cobo, R., Barthel, M., Six, J., Bode, S., Boeckx, P., and Goethals, P.: Greenhouse gas dynamics in an urbanized river system: influence of water quality and land use, Environ. Sci. Pollut. R., 29, 37277–37290, https://doi.org/10.1007/s11356-021-18081-2, 2022.
Hope, D., Palmer, S. M., Billett, M. F., and Dawson, J. J. C.: Carbon dioxide and methane evasion from a temperate peatland stream, Limnol. Oceanogr., 46, 847–857, https://doi.org/10.4319/lo.2001.46.4.0847, 2001.
Hope, D., Palmer, S. M., Billett, M. F., and Dawson, J. J. C.: Variations in dissolved CO2 and CH4 in a first-order stream and catchment: an investigation of soil-stream linkages, Hydrol. Process., 18, 3255–3275, https://doi.org/10.1002/hyp.5657, 2004.
Hu, B., Wang, D., Zhou, J., Meng, W., Li, C., Sun, Z., Guo, X., and Wang, Z.: Greenhouse gases emission from the sewage draining rivers, Sci. Total Environ., 612, 1454–1462, https://doi.org/10.1016/j.scitotenv.2017.08.055, 2018.
Hu, J.: Greenhouse gas emissions and spatiotemporal variation from rivers in the Zoige Plateau, MS thesis, Northwest Agriculture & Forestry University, Xianyang, China, 56 pp., https://kns.cnki.net/KCMS/detail/detail.aspx?dbname=CMFD201601&filename=1015333482.nh (last access: 12 June 2023), 2015.
Huang, Y., Yasarer, L. M. W., Li, Z., Sturm, B. S. M., Zhang, Z., Guo, J., and Shen, Y.: Air–water CO2 and CH4 fluxes along a river–reservoir continuum: Case study in the Pengxi River, a tributary of the Yangtze River in the Three Gorges Reservoir, China, Environ. Monit. Assess., 189, 223, https://doi.org/10.1007/s10661-017-5926-2, 2017.
Huotari, J., Nykänen, H., Forsius, M., and Arvola, L.: Effect of catchment characteristics on aquatic carbon export from a boreal catchment and its importance in regional carbon cycling, Glob. Change Biol., 19, 3607–3620, https://doi.org/10.1111/gcb.12333, 2013.
Hutchins, R. H. S., Prairie, Y. T., and del Giorgio, P. A.: Large-scale landscape drivers of CO2, CH4, DOC, and DIC in boreal river networks, Global Biogeochem. Cy., 33, 125–142, https://doi.org/10.1029/2018GB006106, 2019.
Hutchins, R. H. S., Tank, S. E., Olefeldt, D., Quinton, W. L., Spence, C., Dion, N., Estop-Aragonés, C., and Mengistu, S. G.: Fluvial CO2 and CH4 patterns across wildfire-disturbed ecozones of subarctic Canada: Current status and implications for future change, Glob. Change Biol., 26, 2304–2319, https://doi.org/10.1111/gcb.14960, 2020a.
Hutchins, R. H. S., Casas-Ruiz, J. P., Prairie, Y. T., and del Giorgio, P. A.: Magnitude and drivers of integrated fluvial network greenhouse gas emissions across the boreal landscape in Québec, Water Res., 173, 115556, https://doi.org/10.1016/j.watres.2020.115556, 2020b.
Hutchins, R. H. S., Prairie, Y. T., and del Giorgio, P. A.: The relative importance of seasonality versus regional and network-specific properties in determining the variability of fluvial CO2, CH4 and dissolved organic carbon across boreal Québec, Aquat. Sci., 83, 72, https://doi.org/10.1007/s00027-021-00830-7, 2021.
Hyvonen, N. P., Huttunen, J. T., Shurpali, N. J., Lind, S. E., Marushchek, M. E., Heito, L., and Martikainen, P. J.: The role of drainage ditches in greenhouse gas emissions and surface leaching losses from a cutaway peatland cultivated with a perennial bioenergy crop, Boreal Environ. Res., 18, 109–126, http://hdl.handle.net/10138/229311 (last access: 12 June 2023), 2013.
Jauhiainen, J. and Silvennoinen, H: Diffusion GHG fluxes at tropical peatland drainage canal water surfaces, Suo, 63, 93–105, http://www.suo.fi/article/9882 (last access: 12 June 2023), 2012.
Jin, H., Yoon, T. K., Begum, M. S., Lee, E.-J., Oh, N.-H., Kang, N., and Park, J.-H.: Longitudinal discontinuities in riverine greenhouse gas dynamics generated by dams and urban wastewater, Biogeosciences, 15, 6349–6369, https://doi.org/10.5194/bg-15-6349-2018, 2018.
Jones, J. B. and Mulholland, P. J.: Influence of drainage basin topography and elevation on carbon dioxide and methane supersaturation of stream water, Biogeochemistry, 40, 57–72, https://doi.org/10.1023/A:1005914121280, 1998a.
Jones, J. B. and Mulholland, P. J.: Methane input and evasion in a hardwood forest stream: Effects of subsurface flow from shallow and deep pathway, Limnol. Oceanogr., 43, 1243–1250, https://doi.org/10.4319/lo.1998.43.6.1243, 1998b.
Jurado, A., Borges, A. V., Pujades, E., Briers, P., Nikolenko, O., Dassargues, A., and Brouyère, S.: Dynamics of greenhouse gases in the river–groundwater interface in a gaining river stretch (Triffoy catchment, Belgium), Hydrogeol. J., 26, 2739–2751, https://doi.org/10.1007/s10040-018-1834-y, 2018.
Juutinen, S., Väliranta, M., Kuutti, V., Laine, A. M., Virtanen, T., Seppä, H., Weckström, J., and Tuittila, E.-S.: Short-term and long-term carbon dynamics in a northern peatland-stream-lake continuum: A catchment approach, J. Geophys. Res.-Biogeo., 118, 171–183, https://doi.org/10.1002/jgrg.20028, 2013.
Karlsson, J., Serikova, S., Vorobyev, S. N., Rocher-Ros, G., Denfeld, B. A., and Pokrovsky, O. S.: Carbon emission from Western Siberian inland waters, Zenodo, https://doi.org/10.5281/ZENODO.4153050, 2020.
Kemenes, A., Forsberg, B. R., and Melack, J. M.: Methane release below a tropical hydroelectric dam, Geophys. Res. Lett., 34, L12809, https://doi.org/10.1029/2007GL029479, 2007.
Kemenes, A., Forsberg, B. R., and Melack, J. M.: LBA-ECO LC-07 Methane and Carbon Dioxide emissions from Balbina Reservoir, Brazil, ORNL DAAC, http://daac.ornl.gov/cgi-bin/dsviewer.pl?ds_id=1143 (last access: 12 June 2023), 2013.
Kemenes, A., Forsberg, B. R., and Melack, J. M.: Downstream emissions of CH4 and CO2 from hydroelectric reservoirs (Tucuruí, Samuel, and Curuá-Una) in the Amazon basin, Inland Waters, 6, 295–302, https://doi.org/10.1080/IW-6.3.980, 2016.
Klaus, M., Geibrink, E., Jonsson, A., Bergström, A.-K., Bastviken, D., Laudon, H., Klaminder, J., and Karlsson, J.: Greenhouse gas emissions from boreal inland waters unchanged after forest harvesting, Biogeosciences, 15, 5575–5594, https://doi.org/10.5194/bg-15-5575-2018, 2018.
Kling, G.: Chemistry from thermokarst impacted soils, lakes, and streams near Toolik Lake Alaska, 2008–2011, Environmental Data Initiative, https://doi.org/10.6073/pasta/2e55d1587290e642938ac1a6caed6ec6, 2013.
Kling, G.: Toolik Inlet discharge data collected in summer 1993, Arctic LTER, Toolik Research Station, Alaska, Environmental Data Initiative, https://doi.org/10.6073/pasta/ae3cf97a2496946fa8ba0cf964271e56, 2016a.
Kling, G.: Toolik Inlet discharge data collected in summer 1994, Arctic LTER, Toolik Research Station, Alaska, Environmental Data Initiative, https://doi.org/10.6073/pasta/8cc384d957477d5ad48e926ed26dc89b, 2016b.
Kling, G.: Toolik Inlet discharge data collected in summer 1995, Arctic LTER, Toolik Research Station, Alaska, Environmental Data Initiative, https://doi.org/10.6073/pasta/20e10e53cc8b68cffbe98ed0b234d26a, 2016c.
Kling, G.: Toolik Inlet discharge data collected in summer 1996, Arctic LTER, Toolik Research Station, Alaska, Environmental Data Initiative, https://doi.org/10.6073/pasta/6e9d9bd807d8ec133e91d0e665a1550d, 2016d.
Kling, G.: Toolik Inlet discharge data collected in summer 1997, Arctic LTER, Toolik Research Station, Alaska, Environmental Data Initiative, https://doi.org/10.6073/pasta/33f027ad109d650964a0a084e5df7b11, 2016e.
Kling, G.: Toolik Inlet discharge data collected in summer 1998, Arctic LTER, Toolik Research Station, Alaska, Environmental Data Initiative, https://doi.org/10.6073/pasta/4b78d41f1462c952140b6d2bd4c5d3e4, 2016f.
Kling, G.: Toolik Inlet discharge data collected in summer 1999, Arctic LTER, Toolik Research Station, Alaska, Environmental Data Initiative, https://doi.org/10.6073/pasta/37c5b37970b78525819480aa7e4db43a, 2016g.
Kling, G.: Toolik Inlet discharge data collected in summer 2000, Arctic LTER, Toolik Research Station, Alaska, Environmental Data Initiative, https://doi.org/10.6073/pasta/48d71932248e540223bd5650902dd7a4, 2016h.
Kling, G.: Toolik Inlet discharge data collected in summer 2001, Arctic LTER, Toolik Research Station, Alaska, Environmental Data Initiative, https://doi.org/10.6073/pasta/4EA8FA2D3B89F4BF2B5DE7B98B6A772C, 2016i.
Kling, G.: Toolik Inlet discharge data collected in summer 2002, Arctic LTER, Toolik Research Station, Alaska, Environmental Data Initiative, https://doi.org/10.6073/pasta/aa535873109be90a8a1cb133b45dbc67, 2016j.
Kling, G.: Toolik Inlet discharge data collected in summer 2003, Arctic LTER, Toolik Research Station, Alaska, Environmental Data Initiative, https://doi.org/10.6073/pasta/07d2ff982627a2a73343c1785358d0a6, 2016k.
Kling, G.: Toolik Inlet discharge data collected in summer 2004, Arctic LTER, Toolik Research Station, Alaska, Environmental Data Initiative, https://doi.org/10.6073/pasta/05f608cdb85f2e558febd0fd399da5cf, 2016l.
Kling, G.: Toolik Inlet discharge data collected in summer 2005, Arctic LTER, Toolik Research Station, Alaska, Environmental Data Initiative, https://doi.org/10.6073/pasta/9dde811179666deedd0ecf911be39f65, 2016m.
Kling, G.: Toolik Inlet discharge data collected in summer 2006, Arctic LTER, Toolik Research Station, Alaska, Environmental Data Initiative, https://doi.org/10.6073/pasta/bd8a06d5dab8691912524db28cc24bcd, 2016n.
Kling, G.: Toolik Inlet discharge data collected in summer 2007, Arctic LTER, Toolik Research Station, Alaska, Environmental Data Initiative, https://doi.org/10.6073/pasta/3af4cbab73c38f76b2829c3abff8f703, 2016o.
Kling, G.: Toolik Inlet discharge data collected in summer 2008, Arctic LTER, Toolik Research Station, Alaska, Environmental Data Initiative, https://doi.org/10.6073/pasta/48e780b581b1071f19c7e5f4b165035d, 2016p.
Kling, G.: Toolik Inlet discharge data collected in summer 2009, Arctic LTER, Toolik Research Station, Alaska, Environmental Data Initiative, https://doi.org/10.6073/pasta/94bb7d7a93a46ab5363033de6ee7d603, 2016q.
Kling, G.: Tussock Watershed stream discharge, electrical conductivity, and temperature measurements from 1992, Environmental Data Initiative, https://doi.org/10.6073/pasta/1e224958e278841f9a7a035007c65f21, 2016r.
Kling, G.: Tussock Watershed stream discharge, electrical conductivity, and temperature measurements from 1993, Environmental Data Initiative, https://doi.org/10.6073/pasta/f14f444ce51fa77d5f577db4cdbb0564, 2016s.
Kling, G.: Tussock Watershed stream discharge, electrical conductivity, and temperature measurements from 1994, Environmental Data Initiative, https://doi.org/10.6073/pasta/88124e3e8b4a8bbbd49fbb64d64b62d3, 2016t.
Kling, G.: Tussock Watershed stream discharge, electrical conductivity, and temperature measurements from 1995, Environmental Data Initiative, https://doi.org/10.6073/pasta/7e79c3adc44e965240f1c9d75ea676fb, 2016u.
Kling, G.: Tussock Watershed stream discharge, electrical conductivity, and temperature measurements from 1996, Environmental Data Initiative, https://doi.org/10.6073/pasta/6bd568dba3bfaa58181cfb8abff4d639, 2016v.
Kling, G.: Tussock Watershed stream discharge, electrical conductivity, and temperature measurements from 1997, Environmental Data Initiative, https://doi.org/10.6073/pasta/4c9e9b2bb4861e73dfeaa6bb5e8fb9cd, 2016w.
Kling, G.: Tussock Watershed stream discharge, electrical conductivity, and temperature measurements from 1999, Environmental Data Initiative, https://doi.org/10.6073/pasta/4b943b5a2de08aca8b7dd48542476f12, 2016x.
Kling, G.: Tussock Watershed stream discharge, electrical conductivity, and temperature measurements from 2000, Environmental Data Initiative, https://doi.org/10.6073/pasta/53a45c5a110f0af13c5ae0ed3154b8ca, 2016y.
Kling, G.: Tussock Watershed stream discharge, electrical conductivity, and temperature measurements from 2002, Environmental Data Initiative, https://doi.org/10.6073/pasta/5c3e5f2495561903c027c6b06544cf70, 2016z.
Kling, G.: Tussock Watershed stream discharge, electrical conductivity, and temperature measurements from 2003, Environmental Data Initiative, https://doi.org/10.6073/pasta/b24b8bb901a4b1b825e09c7ab494b39d, 2016aa.
Kling, G.: Tussock Watershed stream discharge, electrical conductivity, and temperature measurements from 2004, Environmental Data Initiative, https://doi.org/10.6073/pasta/459c62f862e1724005eb7d91648bfb44, 2016ab.
Kling, G.: Biogeochemistry data set for Imnavait Creek Weir on the North Slope of Alaska 2002-2018, Environmental Data Initiative, https://doi.org/10.6073/pasta/733c73c6ebffeaec6970b2b0f4dddfe6, 2019a.
Kling, G.: Biogeochemistry data set for soil waters, streams, and lakes near Toolik on the North Slope of Alaska, Environmental Data Initiative, https://doi.org/10.6073/pasta/574fd24522eee7a0c07fc260ccc0e2fa, 2019b.
Kling, G.: Toolik Lake Inlet discharge data collected during summers of 2010 to 2018, Arctic LTER, Toolik Research Station, Alaska, Environmental Data Initiative, https://doi.org/10.6073/pasta/169d1bae55373c44a368727573ef70eb, 2019c.
Kling, G.: Biogeochemistry data set for soil waters, streams, and lakes near Toolik Lake on the North Slope of Alaska, 2012 through 2020 ver. 2, Environmental Data Initiative, https://doi.org/10.6073/pasta/4e25db9ae9372f5339f2795792814845, 2022.
Kling, G. W., Kipphut, G. W., and Miller, M. C.: The flux of CO2 and CH4 from lakes and rivers in arctic Alaska, Hydrobiologia, 240, 23–36, https://doi.org/10.1007/BF00013449, 1992.
Köhn, D., Welpelo, C., Günther, A., and Jurasinski, G.: Drainage ditches contribute considerably to the CH4 budget of a drained and a rewetted temperate fen, Wetlands, 41, 71, https://doi.org/10.1007/s13157-021-01465-y, 2021.
Kokic, J., Sahlée, E., Sobek, S., Vachon, D., and Wallin, M. B.: High spatial variability of gas transfer velocity in streams revealed by turbulence measurements, Inland Waters, 8, 461–473, https://doi.org/10.1080/20442041.2018.1500228, 2018.
Koné, Y. J. M., Abril, G., Delille, B., and Borges, A. V.: Seasonal variability of methane in the rivers and lagoons of Ivory Coast (West Africa), Biogeochemistry, 100, 21–37, https://doi.org/10.1007/s10533-009-9402-0, 2010.
Krickov, I. V., Serikova, S., Pokrovsky, O. S., Vorobyev, S. N., Lim, A. G., Siewert, M. B., and Karlsson, J.: Dataset for Ob River floodplain, Zenodo, https://doi.org/10.5281/ZENODO.4563905, 2021a.
Krickov, I. V., Serikova, S., Pokrovsky, O. S., Vorobyev, S. N., Lim, A. G., Siewert, M. B., and Karlsson, J.: Sizable carbon emission from the floodplain of Ob River, Ecol. Indic., 131, 108164, https://doi.org/10.1016/j.ecolind.2021.108164, 2021b.
Kuhn, C., Bettigole, C., Glick, H. B., Seegmiller, L., Oliver, C. D., and Raymond, P.: Patterns in stream greenhouse gas dynamics from mountains to plains in northcentral Wyoming: Aquatic GHG emissions in Wyoming, J. Geophys. Res.-Biogeo., 122, 2173–2190, https://doi.org/10.1002/2017JG003906, 2017.
LaboMaranger: LaboMaranger/RdN_GHG: data available, Zenodo, https://doi.org/10.5281/ZENODO.4566377, 2021.
Laini, A., Bartoli, M., Castaldi, S., Viaroli, P., Capri, E., and Trevisan, M.: Greenhouse gases (CO2, CH4 and N2O) in lowland springs within an agricultural impacted watershed (Po River Plain, northern Italy), Chem. Ecol., 27, 177–187, https://doi.org/10.1080/02757540.2010.547489, 2011.
Lamarche-Gagnon, G., Wadham, J. L., Sherwood Lollar, B., Arndt, S., Fietzek, P., Beaton, A. D., Tedstone, A. J., Telling, J., Bagshaw, E. A., Hawkings, J. R., Kohler, T. J., Zarsky, J. D., Mowlem, M. C., Anesio, A. M., and Stibal, M.: Greenland melt drives continuous export of methane from the ice-sheet bed, Nature, 565, 73–77, https://doi.org/10.1038/s41586-018-0800-0, 2019.
Lamontagne, R. A., Swinnerton, J. W., Linnenbom, V. J., and Smith, W. D.: Methane concentrations in various marine environments, J. Geophys. Res., 78, 5317–5324, https://doi.org/10.1029/JC078i024p05317, 1973.
Leng, P., Kamjunke, N., Li, F., and Koschorreck, M.: Long-term monitoring CO2 and CH4 concentrations from two German streams, figshare, https://doi.org/10.6084/M9.FIGSHARE.12866945.V1, 2020.
Leng, P., Kamjunke, N., Li, F., and Koschorreck, M.: Temporal patterns of methane emissions from two streams with different riparian connectivity, J. Geophys. Res.-Biogeo., 126, e2020JG006104, https://doi.org/10.1029/2020jg006104, 2021.
Li, L., Yan, R., and Xue, B.: Methane levels of a river network in Wuxi City, China and response to water governance, Water, 12, 2617, https://doi.org/10.3390/w12092617, 2020a.
Li, X., Yao, H., Yu, Y., Cao, Y., and Tang, C.: Greenhouse gases in an urban river: Trend, isotopic evidence for underlying processes, and the impact of in-river structures, J. Hydrol., 591, 125290, https://doi.org/10.1016/j.jhydrol.2020.125290, 2020b.
Liang, X., Zhang, X., Sun, Q., He, C., Chen, X., Liu, X., and Chen, Z.: The role of filamentous algae Spirogyra spp. in methane production and emissions in streams, Aquat. Sci., 78, 227–239, https://doi.org/10.1007/s00027-015-0419-2, 2016.
Lilley, M. D., de Angelis, M. A., and Olson, E. J.: Methane concentrations and estimated fluxes from Pacific Northwest rivers, SIL Communications, 1953–1996, 25, 187–196, https://doi.org/10.1080/05384680.1996.11904080, 1996.
Liu, K., Hu, Z., Wei, X., Jiang, Z., Lu, H., and Wang, C.: Research on methane flux production flux in urban black and odourous rivers in summer: Taking Chaoyang Creek in Nanning City as an example, Earth Environ., 43, 415–419, https://doi.org/10.14050/j.cnki1672-9250/2015.04.005, 2015.
Loken, L., Crawford, J., Stanley, E., Butman, D., and Striegl, R.: Columbia River spatial water chemistry, Environmental Data Initiative, https://doi.org/10.6073/pasta/e881070c9e8f6b7f774d3c65b27a9f69, 2018a.
Loken, L., Crawford, J., and Stanley, E.: Mississippi River spatial water chemistry Environmental Research Letters datasets, Environmental Data Initiative, https://doi.org/10.6073/pasta/c1b9dbd9a96edfb5e39a94cfef2982b9, 2018b.
Looman, A., Maher, D. T., Pendall, E., Bass, A., and Santos, I. R.: The carbon dioxide evasion cycle of an intermittent first-order stream: contrasting water–air and soil–air exchange, Biogeochemistry, 132, 87–102, https://doi.org/10.1007/s10533-016-0289-2, 2017.
Looman, A., Santos, I. R., Tait, D. R., Webb, J., Holloway, C., and Maher, D. T.: Dissolved carbon, greenhouse gases, and δ13C dynamics in four estuaries across a land use gradient, Aquat. Sci., 81, 22, https://doi.org/10.1007/s00027-018-0617-9, 2019.
Lottig, N. and Stanley, E.: North Temperate Lakes LTER: Northern Highlands stream chemistry survey 2006 ver. 18, Environmental Data Initiative, https://doi.org/10.6073/pasta/a73255a67041a580601711c9ac761f26, 2022.
Lottig, N. R., Stanley, E. H., and Maxted, J. T.: Assessing the influence of upstream drainage lakes on fluvial organic carbon in a wetland-rich region, J. Geophys. Res., 117, G03011, https://doi.org/10.1029/2012JG001983, 2012.
Ludwig, S., Holmes, R. M., Natali, S., Mann, P., Schade, J., Jimmie, J., Bristol, E., Peter, D., and Dabrowski, J.: Polaris Project 2017: Aquatic isotopes, carbon, and nitrogen, Yukon-Kuskokwim Delta, Alaska, Arctic Data Center, https://doi.org/10.18739/A20298, 2018a.
Ludwig, S., Holmes, R. M., Natali, S., Schade, J., and Mann, P.: Yukon–Kuskokwim Delta fire: aquatic data, Yukon–Kuskokwim Delta Alaska, 2015–2016, Arctic Data Center, https://doi.org/10.18739/A2HG45, 2018b.
Ludwig, S. M., Natali, S. M., Mann, P. J., Schade, J. D., Holmes, R. M., Powell, M., Fiske, G., and Commane, R.: Using machine learning to predict inland aquatic CO2 and CH4 concentrations and the effects of wildfires in the Yukon–Kuskokwim Delta, Alaska, Global Biogeochem. Cy., 36, e2021GB007146, https://doi.org/10.1029/2021GB007146, 2022.
Ma, P., Li, Y., Qi, L., Tian, X., and Yang, L.: Characteristics of CH4 emission from Shaying River and its influence factors, Environ. Sci. Technol. (China), 40, 26–30, https://doi.org/10.3969/j.issn.1003-6504.2017.02.005, 2017.
Mach, V., Bednařík, A., Čáp, L., Šipoš, J., and Rulík, M.: Seasonal measurement of greenhouse gas concentrations and emissions along the longitudinal profile of a small stream, Pol. J. Environ. Stud., 25, 2047–2056, https://doi.org/10.15244/pjoes/61668, 2016.
Maeck, A., DelSontro, T., McGinnis, D. F., Fischer, H., Flury, S., Schmidt, M., Fietzek, P., and Lorke, A.: Sediment trapping by dams creates methane emission hot spots, Environ. Sci. Technol., 47, 8130–8137, https://doi.org/10.1021/es4003907, 2013.
Maier, M.-S., Teodoru, C. R., and Wehrli, B.: Spatio-temporal variations of lateral and atmospheric carbon fluxes from the Danube Delta (dataset): A 2-year dataset of measured concentrations and fluxes, ETH Research Collection, https://doi.org/10.3929/ethz-b-000416925, 2020.
Maier, M.-S., Teodoru, C. R., and Wehrli, B.: Spatio-temporal variations in lateral and atmospheric carbon fluxes from the Danube Delta, Biogeosciences, 18, 1417–1437, https://doi.org/10.5194/bg-18-1417-2021, 2021.
Manning, C. C., Preston, V. L., Jones, S. F., Michel, A. P. M., Nicholson, D. P., Duke, P. J., Ahmed, M. M. M., Manganini, K., Else, B. G. T., and Tortell, P. D.: River inflow dominates methane emissions in an Arctic coastal system, Geophys. Res. Lett., 47, e2020GL087669, https://doi.org/10.1029/2020gl087669, 2020.
Manning, C. C. M., Preston, V. L, Jones, S. F, Michel, A. P. M., Nicholson, D. P., Duke, P. J., Ahmed, M. M. M., Manganini, K., Else, B. G. T., and Tortell, P. D.: Dissolved methane and nitrous oxide concentrations measured on discrete bottle samples in Cambridge Bay, Nunavut, Canada from 2017, PANGAEA, https://doi.org/10.1594/PANGAEA.907149, 2019a.
Manning, C. C. M., Preston, V. L, Jones, S. F, Michel, A. P. M., Nicholson, D. P., Duke, P. J., Ahmed, M. M. M., Manganini, K., Else, B. G. T., and Tortell, P. D.: Dissolved methane and nitrous oxide concentrations measured on discrete bottle samples in Cambridge Bay, Nunavut, Canada from 2018, PANGAEA, https://doi.org/10.1594/PANGAEA.907150, 2019b.
Manning, F. C.: Carbon dynamics in oil palm agro-ecosystems, PhD thesis, University of Aberdeen, Aberdeen, UK, 435 pp., https://abdn.alma.exlibrisgroup.com/discovery/delivery/44ABE_INST:44ABE_VU1/12152929810005941 (last access: 12 June 2023), 2019a.
Manning, F. C., Kho, L. K., Hill, T. C., Cornulier, T., and Teh, Y. A.: Carbon emissions from oil palm plantations on peat soil, Front. For. Glob. Change, 2, 37, https://doi.org/10.3389/ffgc.2019.00037, 2019b.
Marescaux, A., Thieu, V., and Garnier, J.: Carbon dioxide, methane and nitrous oxide emissions from the human-impacted Seine watershed in France, Sci. Total Environ., 643, 247–259, https://doi.org/10.1016/j.scitotenv.2018.06.151, 2018.
Martin, J. B., Pain, A. J., Martin, E. E., Rahman, S., and Ackerman, P.: Comparisons of nutrients exported from Greenlandic glacial and deglaciated watersheds, Global Biogeochem. Cy., 34, e2020GB006661, https://doi.org/10.1029/2020GB006661, 2020.
Martinez-Cruz, K., Gonzalez-Valencia, R., Sepulveda-Jauregui, A., Plascencia-Hernandez, F., Belmonte-Izquierdo, Y., and Thalasso, F.: Methane emission from aquatic ecosystems of Mexico City, Aquat. Sci., 79, 159–169, https://doi.org/10.1007/s00027-016-0487-y, 2017.
Marwick, T. R., Tamooh, F., Ogwoka, B., Teodoru, C., Borges, A. V., Darchambeau, F., and Bouillon, S.: Dynamic seasonal nitrogen cycling in response to anthropogenic N loading in a tropical catchment, Athi–Galana–Sabaki River, Kenya, Biogeosciences, 11, 443–460, https://doi.org/10.5194/bg-11-443-2014, 2014.
Marwick, T. R., Tamooh, F., Ogwoka, B., Borges, A. V., Darchambeau, F., and Bouillon, S.: A comprehensive biogeochemical record and annual flux estimates for the Sabaki River (Kenya), Biogeosciences, 15, 1683–1700, https://doi.org/10.5194/bg-15-1683-2018, 2018.
Matoušů, A., Rulík, M., Tušer, M., Bednařík, A., Šimek, K., and Bussmann, I.: Methane dynamics in a large river: a case study of the Elbe River, Aquat. Sci., 81, 12, https://doi.org/10.1007/s00027-018-0609-9, 2019.
Matveev, A., Blais, M. A., Laurion, I., and Vincent, W. F.: Dissolved methane, carbon dioxide and limnological data from subarctic rivers, northern Québec, Canada, v. 1.0 (2019–2019), Nordicana D, https://doi.org/10.5885/45660CE-8B92339884C146D0, 2020.
McGinnis, D. F., Bilsley, N., Schmidt, M., Fietzek, P., Bodmer, P., Premke, K., Lorke, A., and Flury, S.: Deconstructing methane emissions from a small northern European river: Hydrodynamics and temperature as key drivers, Environ. Sci. Technol., 50, 11680–11687, https://doi.org/10.1021/acs.est.6b03268, 2016.
Mei, D., Ni, M., Liang, X., Hou, L., Wang, F., and He, C.: Filamentous green algae Spirogyra regulates methane emissions from eutrophic rivers, Environ. Sci. Pollut. R., 28, 3660–3671, https://doi.org/10.1007/s11356-020-10754-8, 2021.
Middelburg, J. J., Nieuwenhuize, J., Iversen, N., Høgh, N., de Wilde, H., Helder, W., Seifert, R., and Christof, O.: Methane distribution in European tidal estuaries, Biogeochemistry, 59, 95–119, https://doi.org/10.1023/A:1015515130419, 2002.
Minkkinen, K. and Laine, J.: Vegetation heterogeneity and ditches create spatial variability in methane fluxes from peatlands drained for forestry, Plant Soil, 285, 289–304, https://doi.org/10.1007/s11104-006-9016-4, 2006.
Minkkinen, K., Laine, J., Nykänen, H., and Martikainen, P. J.: Importance of drainage ditches in emissions of methane from mires drained for forestry, Can. J. For. Res., 27, 949–952, https://doi.org/10.1139/x97-016, 1997.
Morozumi, T., Shingubara, R., Murase, J., Nagai, S., Kobayashi, H., Takano, S., Tei, S., Fan, R., Maximov, T. C., and Sugimoto, A.: Usability of water surface reflectance for the determination of riverine dissolved methane during extreme flooding in northeastern Siberia, Polar Sci., 21, 186–194, https://doi.org/10.1016/j.polar.2019.01.005, 2019.
Morrice, J. A., Dahm, C. N., Valett, H. M., Unnikrishna, P. V., and Campana, M. E.: Terminal electron accepting processes in the alluvial sediments of a headwater stream, J. N. Am. Benthol. Soc., 19, 593–608, https://doi.org/10.2307/1468119, 2000.
Mosher, J., Fortner, A., Phillips, J., Bevelhimer, M., Stewart, A., and Troia, M.: Spatial and temporal correlates of greenhouse gas diffusion from a hydropower reservoir in the southern United States, Water, 7, 5910–5927, https://doi.org/10.3390/w7115910, 2015.
Mulholland, P. J., Helton, A. M., Poole, G. C., Hall, R. O., Hamilton, S. K., Peterson, B. J., Tank, J. L., Ashkenas, L. R., Cooper, L. W., Dahm, C. N., Dodds, W. K., Findlay, S. E. G., Gregory, S. V., Grimm, N. B., Johnson, S. L., McDowell, W. H., Meyer, J. L., Valett, H. M., Webster, J. R., Arango, C. P., Beaulieu, J. J., Bernot, M. J., Burgin, A. J., Crenshaw, C. L., Johnson, L. T., Niederlehner, B. R., O'Brien, J. M., Potter, J. D., Sheibley, R. W., Sobota, D. J., and Thomas, S. M.: Stream denitrification across biomes and its response to anthropogenic nitrate loading, Nature, 452, 202–205, https://doi.org/10.1038/nature06686, 2008.
Murphy, J. F., Arnold, A., Duerdoth, C. P., Hawczak, A., Pacioglu, O., Pretty, J. L., and Jones, J. I.: Temporal variation in temperature and light availability in the Hampshire Avon, United Kingdom [Macronutrient Cycling], NERC Environmental Information Data Centre, https://doi.org/10.5285/9B6A6233-85AD-44F4-BA83-4905B8C48713, 2017.
National Ecological Observatory Network (NEON): Chemical properties of surface water (DP1.20093.001): RELEASE-2021 (RELEASE-2021), NEON, https://doi.org/10.48443/05K7-E011, 2021a.
National Ecological Observatory Network (NEON): Discharge field collection (DP1.20048.001): RELEASE-2021 (RELEASE-2021), NEON, https://doi.org/10.48443/8TD9-4Z94, 2021b.
Neu, V., Neill, C., and Krusche, A. V.: Gaseous and fluvial carbon export from an Amazon forest watershed, Biogeochemistry, 105, 133–147, https://doi.org/10.1007/s10533-011-9581-3, 2011.
Okuku, E. O., Bouillon, S., Tole, M., and Borges, A. V.: Diffusive emissions of methane and nitrous oxide from a cascade of tropical hydropower reservoirs in Kenya, Lakes & Reserv., 24, 127–135, https://doi.org/10.1111/lre.12264, 2019.
Olid, C., Rodellas, V., Rocher-Ros, G., Garcia-Orellana, J., Diego-Feliu, M., Alorda-Kleinglass, A., Bastviken, D., and Karlsson, J.: Groundwater discharge as a driver of methane emissions from Arctic lakes, Nat. Commun., 13, 3667, https://doi.org/10.1038/s41467-022-31219-1, 2022.
Osudar, R., Liebner, S., Alawi, M., Yang, S., Bussmann, I., and Wagner, D.: Methane turnover and methanotrophic communities in arctic aquatic ecosystems of the Lena Delta, Northeast Siberia, FEMS Microbiol. Ecol., 92, fiw116, https://doi.org/10.1093/femsec/fiw116, 2016.
Oviedo-Vargas, D., Genereux, D. P., Dierick, D., and Oberbauer, S. F.: The effect of regional groundwater on carbon dioxide and methane emissions from a lowland rainforest stream in Costa Rica, J. Geophys. Res.-Biogeo., 120, 2579–2595, https://doi.org/10.1002/2015JG003009, 2015.
Pain, A., Martin, J., Martin, E., and Rahman, S.: Hydrogeochemistry of Greenlandic proglacial and nonglacial streams, 2017–2018, Arctic Data Center, https://doi.org/10.18739/A2PC2T94T, 2019.
Peacock, M.: Wicken Lode data, Swedish Agricultural University, Uppsala, Sweden, unpublished, 2020.
Peacock, M.: Forest ditch GHG data, figshare, https://doi.org/10.6084/m9.figshare.15152253.V3, 2021a.
Peacock, M.: Peacock et al., 2021, GCB data, figshare, https://doi.org/10.6084/m9.figshare.14784852.V2, 2021b.
Peacock, M., Ridley, L. M., Evans, C. D., and Gauci, V.: Management effects on greenhouse gas dynamics in fen ditches, Sci. Total Environ., 578, 601–612, https://doi.org/10.1016/j.scitotenv.2016.11.005, 2017.
Peacock, M., Gauci, V., Baird, A. J., Burden, A., Chapman, P. J., Cumming, A., Evans, J. G., Grayson, R. P., Holden, J., Kaduk, J., Morrison, R., Page, S., Pan, G., Ridley, L. M., Williamson, J., Worrall, F., and Evans, C. D.: The full carbon balance of a rewetted cropland fen and a conservation-managed fen, Agr. Ecosyst. Environ., 269, 1–12, https://doi.org/10.1016/j.agee.2018.09.020, 2019.
Peacock, M., Audet, J., Bastviken, D., Cook, S., Evans, C. D., Grinham, A., Holgerson, M. A., Högbom, L., Pickard, A. E., Zieliński, P., and Futter, M. N.: Small artificial waterbodies are widespread and persistent emitters of methane and carbon dioxide, Glob. Change Biol., 27, 5109–5123, https://doi.org/10.1111/gcb.15762, 2021a.
Peacock, M., Granath, G., Wallin, M. B., Högbom, L., and Futter, M. N.: Significant emissions from forest drainage ditches—An unaccounted term in anthropogenic greenhouse gas inventories?, J. Geophys. Res.-Biogeo., 126, e2021JG006478, https://doi.org/10.1029/2021JG006478, 2021b.
Pickard, A., Dinsmore, K. J., Billett, M. F., and Branagan, M.: Aquatic carbon and greenhouse gas concentrations in headwater streams draining from natural, drained and restored peatland catchments in the Flow Country, Scotland, September 2008–August 2010, NERC Environmental Information Data Centre, https://doi.org/10.5285/7525088d-e504-456a-bc55-e48d8ca85303, 2021.
Pickard, A. E., Skiba, U. M., Carvalho, L., Heal, K. V., Rees, R. M., and Harley, J. F.: River Tay, Scotland, water chemistry and greenhouse gas measurements 2009–2010, NERC Environmental Information Data Centre, https://doi.org/10.5285/a61da7da-b7ef-40b7-a324-c3711ef81207, 2019.
Pickard, A. E., Branagan, M., Billett, M. F., Andersen, R., and Dinsmore, K. J.: Effects of peatland management on aquatic carbon concentrations and fluxes, Biogeosciences, 19, 1321–1334, https://doi.org/10.5194/bg-19-1321-2022, 2022.
Pulliam, W. M.: Carbon dioxide and methane exports from a southeastern floodplain swamp, Ecol. Monogr., 63, 29–53, https://doi.org/10.2307/2937122, 1993.
Qin, X., Li, Y., Wan, Y., Fan, M., Liao, Y., Li, Y., Wang, B., and Gao, Q.: Diffusive flux of CH4 and N2O from agricultural river networks: Regression tree and importance analysis, Sci. Total Environ., 717, 137244, https://doi.org/10.1016/j.scitotenv.2020.137244, 2020a.
Qin, X., Li, Y., Wan, Y., Fan, M., Liao, Y., Li, Y., Wang, B., Gao, Q., Wu, H., and Chen, X.: Multiple stable isotopic signatures corroborate the predominance of acetoclastic methanogenesis during CH4 formation in agricultural river networks, Agr. Ecosyst. Environ., 296, 106930, https://doi.org/10.1016/j.agee.2020.106930, 2020b.
Qin, Y., Wang, Z., Li, Z., and Yang, B.: CO2 and CH4 flux across water–air interface and environmental factors in Pengxi River of the Three Gorges Reservoir, J. Earth Environ., 10, 177–189, https://doi.org/10.7515./JEE182071, 2019a.
Qin, Y., Wang, Z., Li, Z., and Yang, B.: CO2 and CH4 partial press and flux across water-air interface in the downstream of the Jinsha River, China, Appl. Ecol. Env. Res., 17, 5823–5839, https://doi.org/10.15666/aeer/1703_58235839, 2019b.
Qu, B., Aho, K. S., Li, C., Kang, S., Sillanpää, M., Yan, F., and Raymond, P. A.: Greenhouse gases emissions in rivers of the Tibetan Plateau, Sci. Rep.-UK, 7, 16573, https://doi.org/10.1038/s41598-017-16552-6, 2017.
Rajkumar, N. A., Barnes, J., Ramesh, R., Purvaja, R., and Upstill-Goddard, R. C.: Methane and nitrous oxide fluxes in the polluted Adyar River and estuary, SE India, Mar. Pollut. Bull., 56, 2043–2051, https://doi.org/10.1016/j.marpolbul.2008.08.005, 2008.
Ran, L., Shi, H., and Yang, X.: Magnitude and drivers of CO2 and CH4 emissions from an arid/semiarid river catchment on the Chinese Loess Plateau, J. Hydrol., 598, 126260, https://doi.org/10.1016/j.jhydrol.2021.126260, 2021.
Reeburgh, W. S., King, J. Y., Regli, S. K., Kling, G. W., Auerbach, N. A., and Walker, D. A.: A CH4 emission estimate for the Kuparuk River basin, Alaska, J. Geophys. Res., 103, 29005–29013, https://doi.org/10.1029/98JD00993, 1998.
Richey, J. E., Devol, A. H., Wofsy, S. C., Victoria, R., and Riberio, M. N. G.: Biogenic gases and the oxidation and reduction of carbon in Amazon River and floodplain waters: Amazon dissolved gases, Limnol. Oceanogr., 33, 551–561, https://doi.org/10.4319/lo.1988.33.4.0551, 1988.
Robison, A. and Wolheim, W.: PIE LTER dissolved methane and water temperature from four headwater streams in Massachusetts and New Hampshire., Environmental Data Initiative, https://doi.org/10.6073/pasta/789147c37923aeecc924ab33b35595eb, 2021a.
Robison, A. and Wollheim, W.: PIE LTER time series of methane, CO2 and N2O ebullition measurements at four headwater streams in Massachusetts and New Hampshire., Environmental Data Initiative, https://doi.org/10.6073/pasta/9b7fb5afa7b55f3c198f37ade701a542, 2021b.
Robison, A. L., Wollheim, W. M., Turek, B., Bova, C., Snay, C., and Varner, R. K.: Spatial and temporal heterogeneity of methane ebullition in lowland headwater streams and the impact on sampling design, Limnol. Oceanogr., 66, 4063–4076, https://doi.org/10.1002/lno.11943, 2021.
Rocher-Ros, G.: Miellajokka summer survey, Swedish University of Agricultural Sciences, Umeå, Sweden, unpublished, 2020.
Rocher-Ros, G.: Impact of reindeer grazing on C emissions and exports from Arctic streams, Swedish University of Agricultural Sciences, Umeå, Sweden, unpublished, 2021.
Roulet, N. T. and Moore, T. R.: The effect of forestry drainage practices on the emission of methane from northern peatlands, Can. J. For. Res., 25, 491–499, https://doi.org/10.1139/x95-055, 1995.
Rovelli, L., Olde, L. A., Heppell, C. M., Binley, A., Yvon-Durocher, G., Glud, R. N., and Trimmer, M.: High-resolution time series of day and night outgassing rates of carbon dioxide and methane for six tributaries of Hampshire River Avon (UK) collected with an automated floating chamber in late spring 2015, figshare, https://doi.org/10.6084/m9.figshare.16545954.v1, 2021a.
Rovelli, L., Olde, L. A., Heppell, C. M., Binley, A., Yvon-Durocher, G., Glud, R. N., and Trimmer, M.: Summary data of chamber-based oxygen and methane consumption and production in the streambed and outgassing of carbon dioxide and methane to the atmosphere collected seasonally for six tributaries of Hampshire River Avon (UK) during 2013–2014, figshare, https://doi.org/10.6084/m9.figshare.16545846.v1, 2021b.
Rovelli, L., Olde, L. A., Heppell, C. M., Binley, A., Yvon-Durocher, G., Glud, R. N., and Trimmer, M.: Contrasting biophysical controls on carbon dioxide and methane outgassing from streams, J. Geophys. Res.-Biogeo., e2021JG006328, 127, https://doi.org/10.1029/2021JG006328, 2022.
Rulík, M., Čáp, L., and Hlaváčová, E.: Methane in the hyporheic zone of a small lowland stream (Sitka, Czech Republic), Limnologica, 30, 359–366, https://doi.org/10.1016/S0075-9511(00)80029-8, 2000.
Sanders, I. A., Heppell, C. M., Cotton, J. A., Wharton, G., Hildrew, A. G., Flowers, E. J., and Trimmer, M.: Emission of methane from chalk streams has potential implications for agricultural practices, Freshwater Biol., 52, 1176–1186, https://doi.org/10.1111/j.1365-2427.2007.01745.x, 2007.
Sansone, F. J., Holmes, M. E., and Popp, B. N.: Methane stable isotopic ratios and concentrations as indicators of methane dynamics in estuaries, Global Biogeochem. Cy., 13, 463–474, https://doi.org/10.1029/1999gb900012, 1999.
Sawakuchi, H. O., Bastviken, D., Sawakuchi, A. O., Krusche, A. V., Ballester, M. V. R., and Richey, J. E.: Methane emissions from Amazonian rivers and their contribution to the global methane budget, Glob. Change Biol., 20, 2829–2840, https://doi.org/10.1111/gcb.12646, 2014.
Sawakuchi, H. O., Bastviken, D., Enrich-Prast, A., Ward, N. D., Camargo, P. B., and Richey, J. E.: Low diffusive methane emissions from the main channel of a large Amazonian run-of-the-river reservoir attributed to high methane oxidation, Front. Environ. Sci., 9, 655455, https://doi.org/10.3389/fenvs.2021.655455, 2021.
Schade, J. D., Bailio, J., and McDowell, W. H.: Greenhouse gas flux from headwater streams in New Hampshire, USA: Patterns and drivers: Greenhouse gas flux from headwater streams, Limnol. Oceanogr., 61, S165–S174, https://doi.org/10.1002/lno.10337, 2016.
Schrier-Uijl, A. P., Kroon, P. S., Leffelaar, P. A., van Huissteden, J. C., Berendse, F., and Veenendaal, E. M.: Methane emissions in two drained peat agro-ecosystems with high and low agricultural intensity, Plant Soil, 329, 509–520, https://doi.org/10.1007/s11104-009-0180-1, 2010.
Schrier-Uijl, A. P., Veraart, A. J., Leffelaar, P. A., Berendse, F., and Veenendaal, E. M.: Release of CO2 and CH4 from lakes and drainage ditches in temperate wetlands, Biogeochemistry, 102, 265–279, https://doi.org/10.1007/s10533-010-9440-7, 2011.
Schuster, P. F. (Ed.): Water and sediment quality in the Yukon River Basin, Alaska, during Water Year 2001, U.S. Geological Survey, Denver, CO, 120 pp., https://doi.org/10.3133/ofr03427, 2003.
Schuster, P. F. (Ed.): Water and sediment quality in the Yukon River Basin, Alaska, during Water Year 2002, U.S. Geological Survey, Denver, CO, 82 pp., https://doi.org/10.3133/ofr20051199, 2006a.
Schuster, P. F. (Ed.): Water and sediment quality in the Yukon River Basin, Alaska, during Water Year 2003, U.S. Geological Survey, Boulder, CO, 74 pp., https://doi.org/10.3133/ofr20051397, 2006b.
Schuster, P. F. (Ed.): Water and sediment quality in the Yukon River Basin, Alaska, during Water Year 2004, U.S. Geological Survey, Boulder, CO, 67 pp., https://doi.org/10.3133/ofr20061258, 2006c.
Schuster, P. F. (Ed.): Water and sediment quality in the Yukon River Basin, Alaska, during Water Year 2005, U.S. Geological Survey, Boulder, CO, 65 pp., https://doi.org/10.3133/ofr20071037, 2007.
Schuster, P. F., Maracle, K. B., and Herman-Mercer, N. (Eds.): Water quality in the Yukon River Basin, Alaska, during Water Years 2006–2008, U.S. Geological Survey, Reston, VA, 220 pp., https://doi.org/10.3133/ofr20101241, 2010.
Selvam, B. P., Natchimuthu, S., Arunachalam, L., and Bastviken, D.: Methane and carbon dioxide emissions from inland waters in India - implications for large scale greenhouse gas balances, Glob. Change Biol., 20, 3397–3407, https://doi.org/10.1111/gcb.12575, 2014.
Shelley, F., Grey, J., and Trimmer, M.: Widespread methanotrophic primary production in lowland chalk rivers, P. R. Soc. B, 281, 20132854, https://doi.org/10.1098/rspb.2013.2854, 2014.
Shi, W., Chen, Q., Yi, Q., Yu, J., Ji, Y., Hu, L., and Chen, Y.: Carbon Emission from Cascade Reservoirs: Spatial heterogeneity and mechanisms, Environ. Sci. Technol., 51, 12175–12181, https://doi.org/10.1021/acs.est.7b03590, 2017.
Sieczko, A. K., Demeter, K., Singer, G. A., Tritthart, M., Preiner, S., Mayr, M., Meisterl, K., and Peduzzi, P.: Aquatic methane dynamics in a human-impacted river-floodplain of the Danube: Floodplain methane emission, Limnol. Oceanogr., 61, S175–S187, https://doi.org/10.1002/lno.10346, 2016.
Silvennoinen, H., Liikanen, A., Rintala, J., and Martikainen, P. J.: Greenhouse gas fluxes from the eutrophic Temmesjoki River and its Estuary in the Liminganlahti Bay (the Baltic Sea), Biogeochemistry, 90, 193–208, https://doi.org/10.1007/s10533-008-9244-1, 2008.
Smith, L. K., Lewis, Jr., W. M., Chanton, J. P., Cronin, G., and Hamilton, S. K.: Methane emissions from the Orinoco River floodplain, Venezuela, Biogeochemistry, 51, 113–140, https://doi.org/10.1023/A:1006443429909, 2000.
Smith, R. L. and Bohlke, J. K.: Methane and nitrous oxide temporal and spatial concentrations in the Iroquois River and Sugar Creek in Northwestern Indiana and Northeastern Illinois, 1999–2003 (ver. 2.0, November 2020), U.S. Geological Survey data release, https://doi.org/10.5066/f7th8kwz, 2018.
Smith, R. L. and Böhlke, J. K.: Methane and nitrous oxide temporal and spatial variability in two midwestern USA streams containing high nitrate concentrations, Sci. Total Environ., 685, 574–588, https://doi.org/10.1016/j.scitotenv.2019.05.374, 2019.
Smith, R. M., Kaushal, S. S., Beaulieu, J. J., Pennino, M. J., and Welty, C.: Influence of infrastructure on water quality and greenhouse gas dynamics in urban streams, Biogeosciences, 14, 2831–2849, https://doi.org/10.5194/bg-14-2831-2017, 2017.
Soja, G., Kitzler, B., and Soja, A.-M.: Emissions of greenhouse gases from Lake Neusiedl, a shallow steppe lake in Eastern Austria, Hydrobiologia, 731, 125–138, https://doi.org/10.1007/s10750-013-1681-8, 2014.
Soued, C. and Prairie, Y. T.: The carbon footprint of a Malaysian tropical reservoir: measured versus modelled estimates highlight the underestimated key role of downstream processes, Biogeosciences, 17, 515–527, https://doi.org/10.5194/bg-17-515-2020, 2020.
Spawn, S., Dunn, S., Fiske, G., Natali, S., Schade, J., and Zimov, N.: Summer methane ebullition from a headwater catchment in Northeastern Siberia, Inland Waters, 5, 224–230, https://doi.org/10.5268/IW-5.3.845, 2015.
Stanley, E. H.: BECWA gases, University of Wisconsin-Madison, USA, unpublished, 2020.
Stets, E. G.: Synoptic carbon gas fluxes from streams, rivers, and lakes in the conterminous US, USGS Landcarbon, 2012 to 2014, U.S. Geological Survey data release, https://www.sciencebase.gov/catalog/item/566f0987e4b09cfe53ca7738, 2016.
Striegl, R. G., Dornblaser, M. M., McDonald, C. P., Rover, J. R., and Stets, E. G.: Carbon dioxide and methane emissions from the Yukon River system, Global Biogeochem. Cy., 26, GB0E05, https://doi.org/10.1029/2012gb004306, 2012.
Sturm, K., Grinham, A., Werner, U., and Yuan, Z.: Sources and sinks of methane and nitrous oxide in the subtropical Brisbane River estuary, South East Queensland, Australia, Estuar. Coast. Shelf S., 168, 10–21, https://doi.org/10.1016/j.ecss.2015.11.002, 2016.
Taillardat, P., Bodmer, P., Deblois, C. P., Ponçot, A., Prijac, A., Riahi, K., Gandois, L., del Giorgio, P. A., Bourgault, M. A., Tremblay, A., and Garneau, M.: Carbon dioxide and methane dynamics in a peatland headwater stream: origin, processes and implications, Zenodo, https://zenodo.org/record/6073957, 2021.
Taillardat, P., Bodmer, P., Deblois, C. P., Ponçot, A., Prijac, A., Riahi, K., Gandois, L., del Giorgio, P. A., Bourgault, M. A., Tremblay, A., and Garneau, M.: Carbon dioxide and methane dynamics in a peatland headwater stream: Origins, processes and implications, J. Geophys. Res.-Biogeo., e2022JG006855, https://doi.org/10.1029/2022JG006855, 2022.
Taniwaki, R. H.: Methane concentrations and fluxes in agricultural and preserved tropical headwater streams, Environmental Data Initiative, https://doi.org/10.6073/pasta/82b1aa19ad5b4ef88939303f79c1e74c, 2022.
Taniwaki, R. H., Cunha, D. G. F., Bento, C. B., Martinelli, L. A., Stanley, E. H., Filoso, S., Ferreira, M. de S., França, M. V., Ribeiro Júnior, J. W., Schiesari, L. C., and do Carmo, J. B.: Methane concentrations and fluxes in agricultural and preserved tropical headwater streams, Sci. Total Environ., 844, 157238, https://doi.org/10.1016/j.scitotenv.2022.157238, 2022.
Teodoru, C. R., Nyoni, F. C., Borges, A. V., Darchambeau, F., Nyambe, I., and Bouillon, S.: Dynamics of greenhouse gases (CO2, CH4, N2O) along the Zambezi River and major tributaries, and their importance in the riverine carbon budget, Biogeosciences, 12, 2431–2453, https://doi.org/10.5194/bg-12-2431-2015, 2015.
Trimmer, M., Hildrew, A. G., Jackson, M. C., Pretty, J. L., and Grey, J.: Evidence for the role of methane-derived carbon in a free-flowing, lowland river food web, Limnol. Oceanogr., 54, 1541–1547, https://doi.org/10.4319/lo.2009.54.5.1541, 2009.
Upstill-Goddard, R. C., Barnes, J., Frost, T., Punshon, S., and Owens, N. J. P.: Methane in the southern North Sea: Low-salinity inputs, estuarine removal, and atmospheric flux, Global Biogeochem. Cy., 14, 1205–1217, https://doi.org/10.1029/1999GB001236, 2000.
Upstill-Goddard, R. C., Salter, M. E., Mann, P. J., Barnes, J., Poulsen, J., Dinga, B., Fiske, G. J., and Holmes, R. M.: The riverine source of CH4 and N2O from the Republic of Congo, western Congo Basin, Biogeosciences, 14, 2267–2281, https://doi.org/10.5194/bg-14-2267-2017, 2017.
Vermaat, J. E., Hellmann, F., Dias, A. T. C., Hoorens, B., van Logtestijn, R. S. P., and Aerts, R.: Greenhouse gas fluxes from Dutch peatland water bodies: Importance of the surrounding landscape, Wetlands, 31, 493–498, https://doi.org/10.1007/s13157-011-0170-y, 2011.
Vidon, P. and Serchan, S.: Impact of stream geomorphology on greenhouse gas concentration in a New York Mountain stream, Water Air Soil Poll., 227, 428, https://doi.org/10.1007/s11270-016-3131-5, 2016.
Villa, J. A., Ju, Y., Smith, G. J., Angle, J. C., Renteria, L., Arntzen, E., Harding, S. F., Stegen, J. C., Wrighton, K. C., and Bohrer, G.: Chamber flux and porewater concentration of CH4, CO2 and N2O, 2018, Columbia River bank at the Hanford site, WA, USA, ESS-DIVE Data Archive, https://doi.org/10.15485/1595105, 2020a.
Villa, J. A., Smith, G. J., Ju, Y., Renteria, L., Angle, J. C., Arntzen, E., Harding, S. F., Ren, H., Chen, X., Sawyer, A. H., Graham, E. B., Stegen, J. C., Wrighton, K. C., and Bohrer, G.: Methane and nitrous oxide porewater concentrations and surface fluxes of a regulated river, Sci. Total Environ., 715, 136920, https://doi.org/10.1016/j.scitotenv.2020.136920, 2020b.
Vorobyev, S. N., Karlsson, J., Kolesnichenko, Y. Y., Korets, M. A., and Pokrovsky, O. S.: Fluvial carbon dioxide emission from the Lena River basin during the spring flood, Biogeosciences, 18, 4919–4936, https://doi.org/10.5194/bg-18-4919-2021, 2021.
Wallin, M. B.: Krycklan catchment time series, Swedish University of Agricultural Sciences, Uppsala, unpublished, 2021.
Wallin, M. B., Löfgren, S., Erlandsson, M., and Bishop, K.: Representative regional sampling of carbon dioxide and methane concentrations in hemiboreal headwater streams reveal underestimates in less systematic approaches: CO2 and CH4 in headwater streams, Global Biogeochem. Cy., 28, 465–479, https://doi.org/10.1002/2013GB004715, 2014.
Wallin, M. B., Campeau, A., Audet, J., Bastviken, D., Bishop, K., Kokic, J., Laudon, H., Lundin, E., Löfgren, S., Natchimuthu, S., Sobek, S., Teutschbein, C., Weyhenmeyer, G. A., and Grabs, T.: Carbon dioxide and methane emissions of Swedish low-order streams-a national estimate and lessons learnt from more than a decade of observations: Carbon dioxide and methane emissions, Limnol. Oceanogr. Lett., 3, 156–167, https://doi.org/10.1002/lol2.10061, 2018.
Wang, D., Chen, Z., Sun, W., Hu, B., and Xu, S.: Methane and nitrous oxide concentration and emission flux of Yangtze Delta plain river net, Sci. China Ser. B, 52, 652–661, https://doi.org/10.1007/s11426-009-0024-0, 2009.
Wang, G., Xia, X., Liu, S., Zhang, L., Zhang, S., Wang, J., Xi, N., and Zhang, Q.: Intense methane ebullition from urban inland waters and its significant contribution to greenhouse gas emissions, Water Res., 189, 116654, https://doi.org/10.1016/j.watres.2020.116654, 2021.
Wang, R., Zhang, H., Zhang, W., Zheng, X., Butterbach-Bahl, K., Li, S., and Han, S.: An urban polluted river as a significant hotspot for water–atmosphere exchange of CH4 and N2O, Environ. Pollut., 264, 114770, https://doi.org/10.1016/j.envpol.2020.114770, 2020.
Wang, X., He, Y., Chen, H., Yuan, X., Peng, C., Yue, J., Zhang, Q., and Zhou, L.: CH4 concentrations and fluxes in a subtropical metropolitan river network: Watershed urbanization impacts and environmental controls, Sci. Total Environ., 622–623, 1079–1089, https://doi.org/10.1016/j.scitotenv.2017.12.054, 2018.
Webb, J. R., Santos, I. R., Maher, D. T., Macdonald, B., Robson, B., Isaac, P., and McHugh, I.: Terrestrial versus aquatic carbon fluxes in a subtropical agricultural floodplain over an annual cycle, Agr. Forest Meteorol., 260–261, 262–272, https://doi.org/10.1016/j.agrformet.2018.06.015, 2018.
Wendt, A. K., Sowers, T., Hynek, S., Lemon, J., Beddings, E., Zheng, G., Li, Z., Williams, J. Z., and Brantley, S. L.: Scientist-nonscientist teams explore methane sources in streams near oil/gas development, J. Contemp. Water Res. Educ., 164, 80–111, https://doi.org/10.1111/j.1936-704X.2018.03286.x, 2018.
Wilcock, R. J. and Sorrell, B. K.: Emissions of greenhouse gases CH4 and N2O from low-gradient streams in agriculturally developed catchments, Water Air Soil Poll., 188, 155–170, https://doi.org/10.1007/s11270-007-9532-8, 2008.
Wilkniss, P. E., Lamontagne, R. A., Larson, R. E., and Swinnerton, J. W.: Atmospheric trace gases and land and sea breezes at the Sepik River coast of Papua New Guinea, J. Geophys. Res., 83, 3672–3674, https://doi.org/10.1029/jc083ic07p03672, 1978.
Woda, J., Wen, T., Lemon, J., Marcon, V., Keeports, C. M., Zelt, F., Steffy, L. Y., and Brantley, S. L.: Methane concentrations in streams reveal gas leak discharges in regions of oil, gas, and coal development, Sci. Total Environ., 737, 140105, https://doi.org/10.1016/j.scitotenv.2020.140105, 2020.
Wu, H., Zhao, Q., Gao, Q., Li, Y., Wan, Y., Li, Y., Tian, D., Liao, Y., Fan, M., Ganjurjav, H. Hu, G., Wang, B., Chen, X., and Qin, X.: Human activities inducing high CH4 diffusive fluxes in an agricultural river catchment in subtropical China, Sustainability, 12, 2114, https://doi.org/10.3390/su12052114, 2020.
Wu, S., Li, S., Zou, Z., Hu, T., Hu, Z., Liu, S., and Zou, J.: High methane emissions largely attributed to ebullitive fluxes from a subtropical river draining a rice paddy watershed in China, Environ. Sci. Technol., 53, 3499–3507, https://doi.org/10.1021/acs.est.8b05286, 2019.
Wu, W.: Characteristics and influencing factors of greenhouse gas emissions from water bodies of Zhongtianshe River Basin in Tianmu Lake area, MS thesis, Nanjing Normal University, Nanjing, China, https://doi.org/10.27245/d.cnki.gnjsu.2020.001588, 2020.
Wu, Y., Li, H., and Chen, W.: Effects and emission characteristics of greenhouse gases from Wenyu River in summer, Environ. Sci. Technol. (China), https://doi.org/10.3969/j.issn.1003-6504.2016.05.002, 39, 8–16, 2016.
Wynn, P.: Direct isotopic evidence of biogenic methane production and efflux from beneath a temperate glacier, Lancaster University, https://doi.org/10.17635/lancaster/researchdata/246, 2018b.
Xiao, Q., Hu, Z., Hu, C., Islam, A. R. M. T., Bian, H., Chen, S., Liu, C., and Lee, X.: A highly agricultural river network in Jurong Reservoir watershed as significant CO2 and CH4 sources, Sci. Total Environ., 769, 144558, https://doi.org/10.1016/j.scitotenv.2020.144558, 2021.
Xu, H., Chen, M., Xiao, S., Yu, Z., and Zheng, X.: Temporal and spatial pattern of dissolved methane concentration in the river of a small karst watershed in Western Hubei, China Environ. Sci., https://kns.cnki.net/kcms/detail/11.2201.x.20210408.0910.002.html (last access: 12 June 2023), 2020.
Yang, L.: Contrasting methane emissions from upstream and downstream rivers and their associated subtropical reservoir in eastern China, Sci. Rep.-UK, 9, 8072, https://doi.org/10.1038/s41598-019-44470-2, 2019.
Yang, L., Li, X., Yan, W., Ma, P., and Wang, J.: CH4 concentrations and emissions from three rivers in the Chaohu Lake watershed in southeast China, J. Integr. Agr., 11, 665–673, https://doi.org/10.1016/S2095-3119(12)60054-9, 2012.
Yang, S.-S., Chen, I.-C., Ching-Pao, L., Liu, L.-Y., and Chang, C.-H.: Carbon dioxide and methane emissions from Tanswei River in Northern Taiwan, Atmos. Pollut. Res., 6, 52–61, https://doi.org/10.5094/APR.2015.007, 2015.
Yavitt, J. B., Lang, G. E., and Sexstone, A. J.: Methane fluxes in wetland and forest soils, beaver ponds, and low-order streams of a temperate forest ecosystem, J. Geophys. Res., 95, 22463, https://doi.org/10.1029/JD095iD13p22463, 1990.
Ye, R., Wu, Q., Zhao, Z., Hu, J., Cui, L., and Ding, H.: Concentrations and emissions of dissolved CH4 and N2O in the Yarlung Tsangpo River, Chinese J. Ecol., 38, 791–798, https://doi.org/10.13292/j.1000-4890.201903.001, 2019.
Yu, Z., Wang, D., Li, Y., Deng, H., Hu, B., Ye, M., Zhou, X., Da, L., Chen, Z., and Xu, S.: Carbon dioxide and methane dynamics in a human-dominated lowland coastal river network (Shanghai, China): CO2 and CH4 in Shanghai River Network, J. Geophys. Res.-Biogeo., 122, 1738–1758, https://doi.org/10.1002/2017JG003798, 2017.
Zhang, F.: Spatio-temporal dynamics of dissolved concentrations of N2O and CH4 and their emission flux in Weihe river of Xinxiang, MS thesis, Henan Normal University, 81 pp., Xinxiang, China, https://kns.cnki.net/KCMS/detail/detail.aspx?dbname=CMFD201701&filename=1016231948.nh (last access: 12 June 2023), 2016.
Zhang, L., Xia, X., Liu, S., Zhang, S., Li, S., Wang, J., Wang, G., Gao, H., Zhang, Z., Wang, Q., Wen, W., Liu, R., Yang, Z., Stanley, E. H., and Raymond, P. A.: Significant methane ebullition from alpine permafrost rivers on the East Qinghai–Tibet Plateau, Nat. Geosci., 13, 349–354, https://doi.org/10.1038/s41561-020-0571-8, 2020.
Zhang, L., Xia, X., Liu, S., Yang, Z., Stanley, E. H., and Raymond, P. A.: A dataset for methane concentrations and fluxes for alpine permafrost streams and rivers on the East Qinghai–Tibet Plateau, Environmental Data Initiative, https://doi.org/10.6073/pasta/3e9ed02d7d89a31eba4c36481255084c, 2021a.
Zhang, L., Xia, X., Battin, T. J., and Stanley, E. H.: Nitrous oxide dataset for East Qinghai–Tibet Plateau waterways, Environmental Data Initiative, https://doi.org/10.6073/pasta/ba9340800403c450e7d942d450237dc4, 2021b.
Zhang, W., Li, H., Pueppke, S. G., and Pang, J.: Restored riverine wetlands in a headwater stream can simultaneously behave as sinks of N2O and hotspots of CH4 production, Environ. Pollut., 284, 117114, https://doi.org/10.1016/j.envpol.2021.117114, 2021a.
Zhang, W., Li, H., Xiao, Q., and Li, X.: Urban rivers are hotspots of riverine greenhouse gas (N2O, CH4, CO2) emissions in the mixed-landscape chaohu lake basin, Water Res., 189, 116624, https://doi.org/10.1016/j.watres.2020.116624, 2021b.
Zhang, Y., Kang, S., Wei, D., Luo, X., Wang, Z., and Gao, T.: Sink or source? Methane and carbon dioxide emissions from cryoconite holes, subglacial sediments, and proglacial river runoff during intensive glacier melting on the Tibetan Plateau, Fundam. Res., 1, 232–239, https://doi.org/10.1016/j.fmre.2021.04.005, 2021.
Zhao, J.: Distribution and fluxes of methane and nitrous oxide in the Changjiang (Yangtze River) and its estuary, MS thesis, Ocean University of China, Qingdao, China, 81 pp., https://kns.cnki.net/KCMS/detail/detail.aspx?dbname=CMFD2012&filename=1011230510.nh (last access: 12 June 2023), 2011.
Zhao, J., Zhang, G.-L., Wu, Y., and Yang, J.: Distribution and emission of methane from the Changjiang, Environ. Sci., 32, 18–25, https://doi.org/10.13227/j.hjkx.2011.01.003, 2011.
Zhao, X.: Study on methane and nitrous oxide emissions from polluted water in Nanjing, MS thesis, Nanjing University of Information Science and Technology, Nanjing, China, 55 pp., https://kns.cnki.net/KCMS/detail/detail.aspx?dbname=CMFD2012&filename=1012369155.nh (last access: 12 June 2023), 2012.
Zolkos, S., Tank, S. E., Striegl, R. G., and Kokelj, S. V.: Thermokarst effects on carbon dioxide and methane fluxes in streams on the Peel Plateau (NWT, Canada), J. Geophys. Res.-Biogeo., 124, 1781–1798, https://doi.org/10.1029/2019JG005038, 2019.
The supplement related to this article is available online at: https://doi.org/10.5194/essd-15-2879-2023-supplement.
EHS conceived of the project idea and led data entry, manuscript preparation, and data curation. LCL developed the code used for unit conversions, was responsible for data conversion and QA/QC, and contributed to data visualization, data analysis, and code curation. GRR was responsible for spatial analyses and contributed to data visualization, code curation, and manuscript preparation. The structure and composition of the paper resulted from collaborative discussions among EHS, GRR, LCL, NJC, SKO, and RAS. All the authors contributed to data acquisition, data entry, data checking, and substantial manuscript revision and editing. Any use of trade, firm, or product names is for descriptive purposes only and does not imply endorsement by the U.S. Government.
The contact author has declared that none of the authors has any competing interests.
Any use of trade, firm, or product names is for descriptive purposes only and does not imply endorsement by the U.S. Government.
Publisher's note: Copernicus Publications remains neutral with regard to jurisdictional claims in published maps and institutional affiliations.
We are grateful to all the authors who generously shared data or made their data public; our science is better for this transparency. This includes the Swedish Infrastructure for Ecosystem Science for collecting and sharing their extensive data included in GRiMeDB. We are also grateful to several of these investigators for patiently and thoughtfully responding to emails from Emily H. Stanley. Corinna Gries provided advice and assistance with data publication. Emily H. Stanley thanks Katrina Forest and Katherine McMahon for guidance during manuscript preparation. Great thanks to Matt Diebel, Simon Topp, Yuanzhi Yao, Karel Castro-Morales, and Bridget Deemer for their thoughtful and detailed comments that improved this paper. The authors were financially supported by the National Science Foundation, NTL LTER (Emily H. Stanley), the Canada Research Chair program (Nora J. Casson), the Swedish Research Council and the Department of Ecology and Environmental Science, Umeå University (Gerard Rocher-Ros), the Swedish Research Council (Vetenskapsrådet), and Svenska Forskningsrådet Formas (Marcus B. Wallin).
This research has been supported by the National Science Foundation (grant no. DEB-2025982, NTL LTER), in part by the Canada Research Chair program, the Swedish Research Council (Vetenskapsrådet) (grant nos. 2021-06667 and 2021-04058), and Svenska Forskningsrådet Formas (grant no. 2019-01105).
This paper was edited by Hao Shi and reviewed by Yuanzhi Yao, Karel Castro-Morales, and Bridget Deemer.
Aho, K. S., Fair, J. H., Hosen, J. D., Kyzivat, E. D., Logozzo, L. A., Rocher-Ros, G., Weber, L. C., Yoon, B., and Raymond, P. A.: Distinct concentration-discharge dynamics in temperate streams and rivers: CO2 exhibits chemostasis while CH4 exhibits source limitation due to temperature control, Limnol. Oceanogr., 66, 3656–3668, https://doi.org/10.1002/lno.11906, 2021.
Allen, G. H. and Pavelsky, T. M.: Global extent of rivers and streams, Science, 361, 585–588, https://doi.org/10.1126/science.aat0636, 2018.
Alshboul, Z., Encinas-Fernández, J., Hofmann, H., and Lorke, A.: Export of dissolved methane and carbon dioxide with effluents from municipal wastewater treatment plants, Environ. Sci. Technol., 50, 5555–5563, https://doi.org/10.1021/acs.est.5b04923, 2016.
Anthony, S. E., Prahl, F. G., and Peterson, T. D.: Methane dynamics in the Willamette River, Oregon, Limnol. Oceanogr., 57, 1517–1530, https://doi.org/10.4319/lo.2012.57.5.1517, 2012.
Attermeyer, K., Casas-Ruiz, J. P., Fuss, T., Pastor, A., Cauvy-Fraunié, S., Sheath, D., Nydahl, A. C., Doretto, A., Portela, A. P., Doyle, B. C., Simov, N., Gutmann Roberts, C., Niedrist, G. H., Timoner, X., Evtimova, V., Barral-Fraga, L., Bašić, T., Audet, J., Deininger, A., Busst, G., Fenoglio, S., Catalán, N., de Eyto, E., Pilotto, F., Mor, J.-R., Monteiro, J., Fletcher, D., Noss, C., Colls, M., Nagler, M., Liu, L., Romero González-Quijano, C., Romero, F., Pansch, N., Ledesma, J. L. J., Pegg, J., Klaus, M., Freixa, A., Herrero Ortega, S., Mendoza-Lera, C., Bednařík, A., Fonvielle, J. A., Gilbert, P. J., Kenderov, L. A., Rulík, M., and Bodmer, P.: Carbon dioxide fluxes increase from day to night across European streams, Commun. Earth Environ., 2, 118, https://doi.org/10.1038/s43247-021-00192-w, 2021.
Barbosa, P. M., Farjalla, V. F., Melack, J. M., Amaral, J. H. F., da Silva, J. S., and Forsberg, B. R.: High rates of methane oxidation in an Amazon floodplain lake, Biogeochemistry, 137, 351–365, https://doi.org/10.1007/s10533-018-0425-2, 2018.
Bastviken, D., Tranvik, L. J., Downing, J. A., Crill, P. M., and Enrich-Prast, A.: Freshwater Methane emissions offset the continental carbon sink, Science, 331, 50–50, https://doi.org/10.1126/science.1196808, 2011.
Baulch, H. M., Dillon, P. J., Maranger, R., and Schiff, S. L.: Diffusive and ebullitive transport of methane and nitrous oxide from streams: Are bubble-mediated fluxes important?, J. Geophys. Res., 116, G04028, https://doi.org/10.1029/2011JG001656, 2011.
Bednařík, A., Blaser, M., Matoušů, A., Hekera, P., and Rulík, M.: Effect of weir impoundments on methane dynamics in a river, Sci. Total Environ., 584–585, 164–174, https://doi.org/10.1016/j.scitotenv.2017.01.163, 2017.
Begum, M. S., Bogard, M. J., Butman, D. E., Chea, E., Kumar, S., Lu, X., Nayna, O. K., Ran, L., Richey, J. E., Tareq, S. M., Xuan, D. T., Yu, R., and Park, J.: Localized pollution impacts on greenhouse gas dynamics in three anthropogenically modified Asian river systems, J. Geophys. Res.-Biogeo., 126, e2020JG006124, https://doi.org/10.1029/2020JG006124, 2021.
Benjamini, Y. and Hochberg, Y.: Controlling the false discovery rate: A practical and powerful approach to multiple testing, J. R. Stat. Soc. B, 57, 289–300, https://doi.org/10.1111/j.2517-6161.1995.tb02031.x, 1995.
Billett, M. F. and Harvey, F. H.: Measurements of CO2 and CH4 evasion from UK peatland headwater streams, Biogeochemistry, 114, 165–181, https://doi.org/10.1007/s10533-012-9798-9, 2013.
Bodmer, P., Vroom, R., Stepina, T., del Giorgio, P., and Kosten, S.: Methane fluxes of vegetated areas in natural freshwater ecosystems: assessments and global significance, Earth ArXiv https://doi.org/10.31223/X5ND0F, 5 December 2021, 2021.
Borges, A. V., Darchambeau, F., Lambert, T., Bouillon, S., Morana, C., Brouyère, S., Hakoun, V., Jurado, A., Tseng, H.-C., Descy, J.-P., and Roland, F. A. E.: Effects of agricultural land use on fluvial carbon dioxide, methane and nitrous oxide concentrations in a large European river, the Meuse (Belgium), Sci. Total Environ., 610–611, 342–355, https://doi.org/10.1016/j.scitotenv.2017.08.047, 2018.
Bouillon, S., Yambélé, A., Spencer, R. G. M., Gillikin, D. P., Hernes, P. J., Six, J., Merckx, R., and Borges, A. V.: Organic matter sources, fluxes and greenhouse gas exchange in the Oubangui River (Congo River basin), Biogeosciences, 9, 2045–2062, https://doi.org/10.5194/bg-9-2045-2012, 2012.
Bretz, K. A., Jackson, A. R., Rahman, S., Monroe, J. M., and Hotchkiss, E. R.: Integrating ecosystem patch contributions to stream corridor carbon dioxide and methane fluxes, J. Geophys. Res.-Biogeo., 126, e2021JG006313, https://doi.org/10.1029/2021JG006313, 2021.
Burns, R., Wynn, P. M., Barker, P., McNamara, N., Oakley, S., Ostle, N., Stott, A. W., Tuffen, H., Zhou, Z., Tweed, F. S., Chesler, A., and Stuart, M.: Direct isotopic evidence of biogenic methane production and efflux from beneath a temperate glacier, Sci. Rep.-UK, 8, 17118. https://doi.org/10.1038/s41598-018-35253-2. 2018.
Bussmann, I., Koedel, U., Schütze, C., Kamjunke, N., and Koschorreck, M.: Spatial variability and hotspots of methane concentrations in a large temperate river, Front. Environ. Sci., 10, 833936, https://doi.org/10.3389/fenvs.2022.833936, 2022.
Call, M., Sanders, C. J., Enrich-Prast, A., Sanders, L., Marotta, H., Santos, I. R., and Maher, D. T.: Radon-traced pore-water as a potential source of CO2 and CH4 to receding black and clear water environments in the Amazon Basin, Limnol. Oceanogr. Lett., 3, 375–383, https://doi.org/10.1002/lol2.10089, 2018.
Campeau, A., Lapierre, J.-F., Vachon, D., and del Giorgio, P. A.: Regional contribution of CO2 and CH4 fluxes from the fluvial network in a lowland boreal landscape of Québec, Global Biogeochem. Cy., 28, 57–69, https://doi.org/10.1002/2013GB004685, 2014.
Campeau, A., Bishop, K., Nilsson, M. B., Klemedtsson, L., Laudon, H., Leith, F. I., Öquist, M., and Wallin, M. B.: Stable carbon isotopes reveal soil-stream DIC linkages in contrasting headwater catchments, J. Geophys. Res.-Biogeo., 123, 149–167, https://doi.org/10.1002/2017JG004083, 2018.
Castro-Morales, K., Canning, A., Körtzinger, A., Göckede, M., Küsel, K., Overholt, W. A., Wichard, T., Redlich, S., Arzberger, S., Kolle, O., and Zimov, N.: Effects of reversal of water flow in an Arctic floodplain river on fluvial emissions of CO2 and CH4, J. Geophys. Res.-Biogeo., 127, e2021JG006485, https://doi.org/10.1029/2021JG006485, 2022.
Chen, S., Wang, D., Ding, Y., Yu, Z., Liu, L., Li, Y., Yang, D., Gao, Y., Tian, H., Cai, R., and Chen, Z.: Ebullition controls on CH4 emissions in an urban, eutrophic river: A potential time-scale bias in determining the aquatic CH4 flux, Environ. Sci. Technol., 55, 7287–7298, https://doi.org/10.1021/acs.est.1c00114, 2021.
Crawford, J. T., Stanley, E. H., Spawn, S. A., Finlay, J. C., Loken, L. C., and Striegl, R. G.: Ebullitive methane emissions from oxygenated wetland streams, Glob. Change Biol., 20, 3408–3422, https://doi.org/10.1111/gcb.12614, 2014.
Crawford, J. T., Dornblaser, M. M., Stanley, E. H., Clow, D. W., and Strieg, R. G.: Source limitation of carbon gas emissions in high-elevation mountain streams and lakes, J. Geophys. Res.-Biogeo., 120, 952–64, https://doi.org/10.1002/2014JG002861, 2015.
Crawford, J. T., Loken, L. C., Stanley, E. H., Stets, E. G., Dornblaser, M. M., and Striegl, R. G.: Basin scale controls on CO2 and CH4 emissions from the Upper Mississippi River, Geophys. Res. Lett., 43, 1973–1979, https://doi.org/10.1002/2015GL067599, 2016.
Crawford, J. T., Loken, L. C., West, W. E., Crary, B., Spawn, S. A., Gubbins, N., Jones, S. E., Striegl, R. G., and Stanley, E. H.: Spatial heterogeneity of within-stream methane concentrations, J. Geophys. Res.-Biogeo., 122, 1036–1048, https://doi.org/10.1002/2016JG003698, 2017.
de Angelis, M. A. and Lilley, M. D.: Methane in surface waters of Oregon estuaries and rivers, Limnol. Oceanogr., 32, 716–722, https://doi.org/10.4319/lo.1987.32.3.0716, 1987.
DelSontro, T., Beaulieu, J. J., and Downing, J. A.: Greenhouse gas emissions from lakes and impoundments: Upscaling in the face of global change, Limnol. Oceanogr. Lett., 3, 64–75, https://doi.org/10.1002/lol2.10073, 2018.
Dowle, M. and Srinivasan, A.: data.table: Extension of 'data.frame', R package version 1.14.2, R Foundation [code], https://CRAN.R-project.org/package=data.table (last access: 8 October 2022), 2021.
Fisher, S. G., Gray, L. J., Grimm, N. B., and Busch, D. E.: Temporal succession in a desert stream ecosystem following flash flooding, Ecol. Monogr., 52, 93–110, https://doi.org/10.2307/2937346, 1982.
Flecker, A. S., Shi, Q., Almeida, R. M., Angarita, H., Gomes-Selman, J. M., García-Villacorta, R., Sethi, S. A., Thomas, S. A., Poff, N. L., Forsberg, B. R., Heilpern, S. A., Hamilton, S. K., Abad, J. D., Anderson, E. P., Barros, N., Bernal, I. C., Bernstein, R., Cañas, C. M., Dangles, O., Encalada, A. C., Fleischmann, A. S., Goulding, M., Higgins, J., Jézéquel, C., Larson, E. I., McIntyre, P. B., Melack, J. M., Montoya, M., Oberdorff, T., Paiva, R., Perez, G., Rappazzo, B. H., Steinschneider, S., Torres, S., Varese, M., Walter, M. T., Wu, X., Xue, Y., Zapata-Ríos, X. E., and Gomes, C. P.: Reducing adverse impacts of Amazon hydropower expansion, Science, 375, 753–760, https://doi.org/10.1126/science.abj4017, 2022.
Gatti, R. C., Callaghan, T. V., Rozhkova-Timina, I., Dudko, A., Lim, A., Vorobyev, S. N., Kirpotin, S. N., and Pokrovsky, O. S.: The role of Eurasian beaver (Castor fiber) in the storage, emission and deposition of carbon in lakes and rivers of the River Ob flood plain, western Siberia, Sci. Total Environ., 644, 1371–1379, https://doi.org/10.1016/j.scitotenv.2018.07.042, 2018.
Gómez-Gener, L., Hotchkiss, E. R., Laudon, H., and Sponseller, R. A.: Integrating discharge-concentration dynamics across carbon forms in a boreal landscape, Water Resour. Res., 57, e2020WR028806, https://doi.org/10.1029/2020WR028806, 2021a.
Gómez-Gener, L., Rocher-Ros, G., Battin, T., Cohen, M. J., Dalmagro, H. J., Dinsmore, K. J., Drake, T. W., Duvert, C., Enrich-Prast, A., Horgby, Å., Johnson, M. S., Kirk, L., Machado-Silva, F., Marzolf, N. S., McDowell, M. J., McDowell, W. H., Miettinen, H., Ojala, A. K., Peter, H., Pumpanen, J., Ran, L., Riveros-Iregui, D. A., Santos, I. R., Six, J., Stanley, E. H., Wallin, M. B., White, S. A., and Sponseller, R. A.: Global carbon dioxide efflux from rivers enhanced by high nocturnal emissions, Nat. Geosci., 14, 289–294, https://doi.org/10.1038/s41561-021-00722-3, 2021b.
Gries, C., Hanson, P. C., O'Brien, M., Servilla, M., Vanderbilt, K., and Waide, R.: The Environmental Data Initiative: Connecting the past to the future through data reuse, Ecol. Evol., 13, e9592, https://doi.org/10.1002/ece3.9592, 2023.
Gurnell, A. M., O'Hare, J. M., O'Hare, M. T., Dunbar, M. J., and Scarlett, P. M.: An exploration of associations between assemblages of aquatic plant morphotypes and channel geomorphological properties within British rivers, Geomorphology, 116, 135–144, https://doi.org/10.1016/j.geomorph.2009.10.014, 2010.
Hall Jr., R. O. and Ulseth, A. J.: Gas exchange in streams and rivers, WIREs Water, 7, e1391, https://doi.org/10.1002/wat2.1391, 2020.
Hlaváčová, E., Rulík, M., Čáp, L., and Mach, V.: Greenhouse gas (CO2, CH4, N2O) emissions to the atmosphere from a small lowland stream in Czech Republic, Arch. Hydrobiol., 165, 339–353, https://doi.org/10.1127/0003-9136/2006/0165-0339, 2006.
Ho, L., Jerves-Cobo, R., Barthel, M., Six, J., Bode, S., Boeckx, P., and Goethals, P.: Greenhouse gas dynamics in an urbanized river system: influence of water quality and land use, Environ. Sci. Pollut. R., 29, 37277–37290, https://doi.org/10.1007/s11356-021-18081-2, 2022.
Hollister, J. W., Robitaille, A. L., Beck, M. W., Johnson, M., and Shah, T.: jhollist/elevatr: CRAN Release 0.4.2, Zenodo [code], https://doi.org/10.5281/zenodo.5809645, 2021.
Horton, R. E.: Erosional development of streams and their drainage basins; hydrophysical approach to quantitative morphology, Geol. Soc. Am. Bull., 56, 275, https://doi.org/10.1130/0016-7606(1945)56[275:EDOSAT]2.0.CO;2, 1945.
Hugelius, G., Strauss, J., Zubrzycki, S., Harden, J. W., Schuur, E. A. G., Ping, C.-L., Schirrmeister, L., Grosse, G., Michaelson, G. J., Koven, C. D., O'Donnell, J. A., Elberling, B., Mishra, U., Camill, P., Yu, Z., Palmtag, J., and Kuhry, P.: Estimated stocks of circumpolar permafrost carbon with quantified uncertainty ranges and identified data gaps, Biogeosciences, 11, 6573–6593, https://doi.org/10.5194/bg-11-6573-2014, 2014.
Hughes, B. B., Beas-Luna, R., Barner, A. K., Brewitt, K., Brumbaugh, D. R., Cerny-Chipman, E. B., Close, S. L., Coblentz, K. E., de Nesnera, K. L., Drobnitch, S. T., Figurski, J. D., Focht, B., Friedman, M., Freiwald, J., Heady, K. K., Heady, W. N., Hettinger, A., Johnson, A., Karr, K. A., Mahoney, B., Moritsch, M. M., Osterback, A.-M. K., Reimer, J., Robinson, J., Rohrer, T., Rose, J. M., Sabal, M., Segui, L. M., Shen, C., Sullivan, J., Zuercher, R., Raimondi, P. T., Menge, B. A., Grorud-Colvert, K., Novak, M., and Carr, M. H.: Long-term studies contribute disproportionately to ecology and policy, BioScience, 67, 271–281, https://doi.org/10.1093/biosci/biw185, 2017.
Hutchins, R. H. S., Casas-Ruiz, J. P., Prairie, Y. T., and del Giorgio, P. A.: Magnitude and drivers of integrated fluvial network greenhouse gas emissions across the boreal landscape in Québec, Water Res., 173, 115556, https://doi.org/10.1016/j.watres.2020.115556, 2020.
IPCC: Climate Change: The Physical Science Basis. Contribution of Working Group I to the Sixth Assessment Report of the Intergovernmental Panel on Climate Change, edited by: Masson-Delmotte, V., Zhai, P., Pirani, A., Connors, S. L., Péan, C., Berger, S., Caud, N., Chen, Y., Goldfarb, L., Gomis, M. I., Huang, M., Leitzell, K., Lonnoy, E., Matthews, J. B. R., Maycock, T. K., Waterfield, T., Yelekçi, O., Yu, R., and Zhou., B., Cambridge University Press, Cambridge, United Kingdom and New York, NY, USA, https://www.ipcc.ch/report/sixth-assessment-report-working-group-i/ (last access: 12 June 2023), 2021.
Jin, H., Yoon, T. K., Begum, M. S., Lee, E.-J., Oh, N.-H., Kang, N., and Park, J.-H.: Longitudinal discontinuities in riverine greenhouse gas dynamics generated by dams and urban wastewater, Biogeosciences, 15, 6349–6369, https://doi.org/10.5194/bg-15-6349-2018, 2018.
Kemenes, A., Forsberg, B. R., and Melack, J. M.: Methane release below a tropical hydroelectric dam, Geophys. Res. Lett., 34, L12809, https://doi.org/10.1029/2007gl029479, 2007.
Kling, G.: Biogeochemistry data set for Imnavait Creek Weir on the North Slope of Alaska 2002-2018, Environmental Data Initiative [data set], https://doi.org/10.6073/pasta/733c73c6ebffeaec6970b2b0f4dddfe6, 2019a.
Kling, G.: Biogeochemistry data set for soil waters, streams, and lakes near Toolik on the North Slope of Alaska, Environmental Data Initiative [data set], https://doi.org/10.6073/pasta/574fd24522eee7a0c07fc260ccc0e2fa, 2019b.
Kling, G.: Biogeochemistry data set for soil waters, streams, and lakes near Toolik Lake on the North Slope of Alaska, 2012 through 2020 ver 2, Environmental Data Initiative, https://doi.org/10.6073/pasta/4e25db9ae9372f5339f2795792814845, 2022.
Kokic, J., Sahlée, E., Sobek, S., Vachon, D., and Wallin, M. B.: High spatial variability of gas transfer velocity in streams revealed by turbulence measurements, Inland Waters, 8, 461–473, https://doi.org/10.1080/20442041.2018.1500228, 2018.
Kuhn, C., Bettigole, C., Glick, H. B., Seegmiller, L., Oliver, C. D., and Raymond, P.: Patterns in stream greenhouse gas dynamics from mountains to plains in northcentral Wyoming, J. Geophys. Res.-Biogeo., 122, 2173–2190, https://doi.org/10.1002/2017jg003906, 2017.
Lamarche-Gagnon, G., Wadham, J. L., Sherwood Lollar, B., Arndt, S., Fietzek, P., Beaton, A. D., Tedstone, A. J., Telling, J., Bagshaw, E. A., Hawkings, J. R., Kohler, T. J., Zarsky, J. D., Mowlem, M. C., Anesio, A. M., and Stibal, M.: Greenland melt drives continuous export of methane from the ice-sheet bed, Nature, 565, 73–77, https://doi.org/10.1038/s41586-018-0800-0, 2019.
Lamontagne, R. A., Swinnerton, J. W., Linnenbom, V. J., and Smith, W. D.: Methane concentrations in various marine environments, J. Geophys. Res., 78, 5317–5324, https://doi.org/10.1029/JC078i024p05317, 1973.
Leng, P., Kamjunke, N., Li, F., and Koschorreck, M.: Temporal patterns of methane emissions from two streams with different riparian connectivity, J. Geophys. Res.-Biogeo., 126, e2020JG006104, https://doi.org/10.1029/2020jg006104, 2021.
Li, M., Peng, C., Zhang, K., Xu, L., Wang, J., Yang, Y., Li, P., Liu, Z., and He, N.: Headwater stream ecosystem: an important source of greenhouse gases to the atmosphere, Water Res., 190, 116738, https://doi.org/10.1016/j.watres.2020.116738, 2021.
Lian, X., Piao, S., Chen, A., Huntingford, C., Fu, B., Li, L. Z. X., Huang, J., Sheffield, J., Berg, A. M., Keenan, T. F., McVicar, T. R., Wada, Y., Wang, X., Wang, T., Yang, Y., and Roderick, M. L.: Multifaceted characteristics of dryland aridity changes in a warming world, Nat. Rev. Earth Environ., 2, 232–250, https://doi.org/10.1038/s43017-021-00144-0, 2021.
Linke, S., Lehner, B., Ouellet Dallaire, C., Ariwi, J., Grill, G., Anand, M., Beames, P., Burchard-Levine, V., Maxwell, S., Moidu, H., Tan, F., and Thieme, M.: Global hydro-environmental sub-basin and river reach characteristics at high spatial resolution, Sci. Data, 6, 283, https://doi.org/10.1038/s41597-019-0300-6, 2019.
Liu, S., Kuhn, C., Amatulli, G., Aho, K., Butman, D. E., Allen, G. H., Lin, P., Pan, M., Yamazaki, D., Brinkerhoff, C., Gleason, C., Xia, X., and Raymond, P. A.: The importance of hydrology in routing terrestrial carbon to the atmosphere via global streams and rivers, P. Natl. Acad. Sci. USA, 119, e2106322119, https://doi.org/10.1073/pnas.2106322119, 2022.
Loken, L. C, Crawford, J. T., Stanley, E. H., Butman, D., and Striegl, R.: Columbia River spatial water chemistry, Environmental Data Initiative [data set], https://doi.org/10.6073/pasta/e881070c9e8f6b7f774d3c65b27a9f69, 2018.
Lorke, A., Bodmer, P., Noss, C., Alshboul, Z., Koschorreck, M., Somlai-Haase, C., Bastviken, D., Flury, S., McGinnis, D. F., Maeck, A., Müller, D., and Premke, K.: Technical note: drifting versus anchored flux chambers for measuring greenhouse gas emissions from running waters, Biogeosciences, 12, 7013–7024, https://doi.org/10.5194/bg-12-7013-2015, 2015.
Lupon, A., Denfeld, B. A., Laudon, H., Leach, J., Karlsson, J., and Sponseller, R. A.: Groundwater inflows control patterns and sources of greenhouse gas emissions from streams, Limnol. Oceanogr., 64, 1545–1557, https://doi.org/10.1002/lno.11134, 2019.
Metcalfe, D. B., Hermans, T. D. G., Ahlstrand, J., Becker, M., Berggren, M., Björk, R. G., Björkman, M. P., Blok, D., Chaudhary, N., Chisholm, C., Classen, A. T., Hasselquist, N. J., Jonsson, M., Kristensen, J. A., Kumordzi, B. B., Lee, H., Mayor, J. R., Prevéy, J., Pantazatou, K., Rousk, J., Sponseller, R. A., Sundqvist, M. K., Tang, J., Uddling, J., Wallin, G., Zhang, W., Ahlström, A., Tenenbaum, D. E., and Abdi, A. M.: Patchy field sampling biases understanding of climate change impacts across the Arctic, Nat. Ecol. Evol., 2, 1443–1448, https://doi.org/10.1038/s41559-018-0612-5, 2018.
Natchimuthu, S., Wallin, M. B., Klemedtsson, L., and Bastviken, D.: Spatio-temporal patterns of stream methane and carbon dioxide emissions in a hemiboreal catchment in Southwest Sweden, Sci. Rep.-UK, 7, 39729, https://doi.org/10.1038/srep39729, 2017.
NOAA: Increase in atmospheric methane set another record during 2021, https://www.noaa.gov/news-release/increase-in-atmospheric-methane-set-another-record-during-2021 (last access: 20 September 2022), 2022.
Park, J.-H., Nayna, O. K., Begum, M. S., Chea, E., Hartmann, J., Keil, R. G., Kumar, S., Lu, X., Ran, L., Richey, J. E., Sarma, V. V. S. S., Tareq, S. M., Xuan, D. T., and Yu, R.: Reviews and syntheses: Anthropogenic perturbations to carbon fluxes in Asian river systems – concepts, emerging trends, and research challenges, Biogeosciences, 15, 3049–3069, https://doi.org/10.5194/bg-15-3049-2018, 2018.
Peacock, M., Audet, J., Bastviken, D., Futter, M. N., Gauci, V., Grinham, A., Harrison, J. A., Kent, M. S., Kosten, S., Lovelock, C. E., Veraart, A. J., and Evans, C. D.: Global importance of methane emissions from drainage ditches and canals, Environ. Res. Lett., 16, 044010, https://doi.org/10.1088/1748-9326/abeb36, 2021.
Pebesma, E.: Simple features for R: Standardized support for spatial vector data, R Journal, 10, 439, https://doi.org/10.32614/RJ-2018-009, 2018.
Pedersen, T. L.: Patchwork: the composer of plots, R package version 1.1.2, R Foundation [code] https://CRAN.R-project.org/package=patchwork (last access: 14 June 2023), 2020.
Rajkumar, N. A., Barnes, J., Ramesh, R., Purvaja, R., and Upstill-Goddard, R. C.: Methane and nitrous oxide fluxes in the polluted Adyar River and estuary, SE India, Mar. Pollut. Bull., 56, 2043–2051, https://doi.org/10.1016/j.marpolbul.2008.08.005, 2008.
Ran, L., Shi, H., and Yang, X.: Magnitude and drivers of CO2 and CH4 emissions from an arid/semiarid river catchment on the Chinese Loess Plateau, J. Hydrol., 598, 126260, https://doi.org/10.1016/j.jhydrol.2021.126260, 2021.
Raymond, P. A., Zappa, C. J., Butman, D., Bott, T. L., Potter, J., Mulholland, P., Laursen, A. E., McDowell, W. H., and Newbold, D.: Scaling the gas transfer velocity and hydraulic geometry in streams and small rivers, Limnol. Oceanogr. Fluids Enviro., 2, 41–53, https://doi.org/10.1215/21573689-1597669, 2012.
R Core Team: R: A language and environment for statistical computing, R Foundation for Statistical Computing [code], Vienna, Austria, https://www.R-project.org/ (last access: 14 June 2023), 2021.
Riis, T. and Biggs, B. J. F.: Hydrologic and hydraulic control of macrophyte establishment and performance in streams, Limnol. Oceanogr., 48, 1488–1497, https://doi.org/10.4319/lo.2003.48.4.1488, 2003.
Robison, A. L., Wollheim, W. M., Turek, B., Bova, C., Snay, C., and Varner, R. K.: Spatial and temporal heterogeneity of methane ebullition in lowland headwater streams and the impact on sampling design, Limnol. Oceanogr., 66, 4063–4076, https://doi.org/10.1002/lno.11943, 2021.
Rocher-Ros, G., Stanley, E. H., Loken, L. C., Casson, N. J., Raymond, P. A., Liu, S., Amantulli, G., and Sponseller, R. A.: Global methane emissions from running waters, Nature, accepted, 2023.
Rosentreter, J. A., Borges, A. V., Deemer, B. R., Holgerson, M. A., Liu, S., Song, C., Melack, J., Raymond, P. A., Duarte, C. M., Allen, G. H., Olefeldt, D., Poulter, B., Battin, T. I., and Eyre, B. D.: Half of global methane emissions come from highly variable aquatic ecosystem sources, Nat. Geosci., 14, 225–230, https://doi.org/10.1038/s41561-021-00715-2, 2021.
Rovelli, L., Olde, L. A., Heppell, C. M., Binley, A., Yvon-Durocher, G., Glud, R. N., and Trimmer, M.: Contrasting biophysical controls on carbon dioxide and methane outgassing from streams, J. Geophys. Res.-Biogeo., 127, e2021JG006328, https://doi.org/10.1029/2021JG006328, 2022.
Sabo, J. L., Sinha, T., Bowling, L. C., Schoups, G. H. W., Wallender, W. W., Campana, M. E., Cherkauer, K. A., Fuller, P. L., Graf, W. L., Hopmans, J. W., Kominoski, J. S., Taylor, C., Trimble, S. W., Webb, R. H., and Wohl, E. E.: Reclaiming freshwater sustainability in the Cadillac Desert, P. Natl. Acad. Sci. USA, 107, 21263–21269, https://doi.org/10.1073/pnas.1009734108, 2010.
Sanders, I. A., Heppell, C. M., Cotton, J. A., Wharton, G., Hildrew, A. G., Flowers, E. J., and Trimmer, M.: Emission of methane from chalk streams has potential implications for agricultural practices, Freshwater Biol., 52, 1176–1186, https://doi.org/10.1111/j.1365-2427.2007.01745.x, 2007.
Saunois, M., Stavert, A. R., Poulter, B., Bousquet, P., Canadell, J. G., Jackson, R. B., Raymond, P. A., Dlugokencky, E. J., Houweling, S., Patra, P. K., Ciais, P., Arora, V. K., Bastviken, D., Bergamaschi, P., Blake, D. R., Brailsford, G., Bruhwiler, L., Carlson, K. M., Carrol, M., Castaldi, S., Chandra, N., Crevoisier, C., Crill, P. M., Covey, K., Curry, C. L., Etiope, G., Frankenberg, C., Gedney, N., Hegglin, M. I., Höglund-Isaksson, L., Hugelius, G., Ishizawa, M., Ito, A., Janssens-Maenhout, G., Jensen, K. M., Joos, F., Kleinen, T., Krummel, P. B., Langenfelds, R. L., Laruelle, G. G., Liu, L., Machida, T., Maksyutov, S., McDonald, K. C., McNorton, J., Miller, P. A., Melton, J. R., Morino, I., Müller, J., Murguia-Flores, F., Naik, V., Niwa, Y., Noce, S., O'Doherty, S., Parker, R. J., Peng, C., Peng, S., Peters, G. P., Prigent, C., Prinn, R., Ramonet, M., Regnier, P., Riley, W. J., Rosentreter, J. A., Segers, A., Simpson, I. J., Shi, H., Smith, S. J., Steele, L. P., Thornton, B. F., Tian, H., Tohjima, Y., Tubiello, F. N., Tsuruta, A., Viovy, N., Voulgarakis, A., Weber, T. S., van Weele, M., van der Werf, G. R., Weiss, R. F., Worthy, D., Wunch, D., Yin, Y., Yoshida, Y., Zhang, W., Zhang, Z., Zhao, Y., Zheng, B., Zhu, Q., Zhu, Q., and Zhuang, Q.: The Global Methane Budget 2000–2017, Earth Syst. Sci. Data, 12, 1561–1623, https://doi.org/10.5194/essd-12-1561-2020, 2020.
Sawakuchi, H. O., Bastviken, D., Sawakuchi, A. O., Krusche, A. V., Ballester, M. V. R., and Richey, J. E.: Methane emissions from Amazonian rivers and their contribution to the global methane budget, Glob. Change Biol., 20, 2829–2840, https://doi.org/10.1111/gcb.12646, 2014.
Smith, R. L. and Böhlke, J. K.: Methane and nitrous oxide temporal and spatial variability in two midwestern USA streams containing high nitrate concentrations, Sci. Total Environ., 685, 574–588, https://doi.org/10.1016/j.scitotenv.2019.05.374, 2019.
Smith, R. M., Kaushal, S. S., Beaulieu, J. J., Pennino, M. J., and Welty, C.: Influence of infrastructure on water quality and greenhouse gas dynamics in urban streams, Biogeosciences, 14, 2831–2849, https://doi.org/10.5194/bg-14-2831-2017, 2017.
Spawn, S., Dunn, S., Fiske, G., Natali, S., Schade, J., and Zimov, N.: Summer methane ebullition from a headwater catchment in Northeastern Siberia, Inland Waters, 5, 224–230, https://doi.org/10.5268/IW-5.3.845, 2015.
Stanley, E. H., Loken, L. C., Crawford, J. T., Casson, N. J, Oliver, S. K, Gries, C., and Christel, S.: A global database of methane concentrations and atmospheric fluxes for streams and rivers, Environmental Data Initiative [data set], https://doi.org/10.6073/pasta/21f5bd6642e9689baf90262f3c85ac4a, 2015.
Stanley, E. H., Casson, N. J., Christel, S. T., Crawford, J. T., Loken, L. C., and Oliver, S. K.: The ecology of methane in streams and rivers: patterns, controls, and global significance, Ecol. Monogr., 86, 146–171, https://doi.org/10.1890/15-1027, 2016.
Stanley, E. H., Collins, S. M., Lottig, N. R., Oliver, S. K., Webster, K. E., Cheruvelil, K. S., and Soranno, P. A.: Biases in lake water quality sampling and implications for macroscale research, Limnol. Oceanogr., 64, 1572–1585, https://doi.org/10.1002/lno.11136, 2019.
Stanley, E. H., Loken, L. C., Rocher-Ros, G., Casson, N. J., Oliver, S. K., Sponseller, R. A., Wallin, M., and Zhang, L.: GRiMeDB: a comprehensive global database of methane concentrations and fluxes in fluvial ecosystems with supporting physical and chemical information, ver 2, Environmental Data Initiative [code/data set], https://doi.org/10.6073/pasta/f48cdb77282598052349e969920356ef, 2023.
Stow, C. A., Webster, K. E., Wagner, T., Lottig, N., Soranno, P. A., and Cha, Y.: Small values in big data: The continuing need for appropriate metadata, Ecol. Inform., 45, 26–30, https://doi.org/10.1016/j.ecoinf.2018.03.002, 2018.
Stringer, L. C., Mirzabaev, A., Benjaminsen, T. A., Harris, R. M. B., Jafari, M., Lissner, T. K., Stevens, N., and Tirado-von der Pahlen, C.: Climate change impacts on water security in global drylands, One Earth, 4, 851–864, https://doi.org/10.1016/j.oneear.2021.05.010, 2021.
Taillardat, P., Bodmer, P., Deblois, C. P., Ponçot, A., Prijac, A., Riahi, K., Gandois, L., del Giorgio, P. A., Bourgault, M. A., Tremblay, A., and Garneau, M.: Carbon dioxide and methane dynamics in a peatland headwater stream: Origins, processes and implications, J. Geophys. Res.-Biogeo., 127, e2022JG006855, https://doi.org/10.1029/2022jg006855, 2022.
Turner, A. J., Frankenberg, C., and Kort, E. A.: Interpreting contemporary trends in atmospheric methane, P. Natl. Acad. Sci. USA, 116, 2805–2813, https://doi.org/10.1073/pnas.1814297116, 2019.
van den Hoogen, J., Robmann, N., Routh, D., Lauber, T., van Tiel, N., Danylo, O., and Crowther, T. W.: A geospatial mapping pipeline for ecologists, BioRxiv, https://doi.org/10.1101/2021.07.07.451145, 9 July 2021.
Wallin, M. B., Campeau, A., Audet, J., Bastviken, D., Bishop, K., Kokic, J., Laudon, H., Lundin, E., Löfgren, S., Natchimuthu, S., Sobek, S., Teutschbein, C., Weyhenmeyer, G. A., and Grabs, T.: Carbon dioxide and methane emissions of Swedish low-order streams – a national estimate and lessons learnt from more than a decade of observations, Limnol. Oceanogr. Lett., 3, 156–167, https://doi.org/10.1002/lol2.10061, 2018.
Wickham, H.: ggplot2: Elegant Graphics for Data Analysis, Springer-Verlag, New York, https://doi.org/10.1007/978-3-319-24277-4, 2016.
Wickham, H., François, R., Henry, L., and Müller, K.: dplyr: A Grammar of Data Manipulation, R package version 1.0.7, R Foundation [code] https://CRAN.R-project.org/package=dplyr (last access: 14 June 2023), 2021.
Wilcock, R. J. and Sorrell, B. K.: Emissions of greenhouse gases CH4 and N2O from low-gradient streams in agriculturally developed catchments, Water Air Soil Poll., 188, 155–170, https://doi.org/10.1007/s11270-007-9532-8, 2008.
Wilkinson, M., Dumontier, M., Aalbersberg, I., Appleton, G., Axton, M., Baak, A., Blomberg, N., Boiten J.-W., Bonino da Silva Santos, L., Bourne, P. E., Bouwman, J., Brookes, A. J., Clark, T., Crosas, M., Dillo, I., Dumon, O., Edmunds, S., Evelo, C. T., Finkers, R., Gonzalez-Beltran, A., Gray, A. J. G., Groth, P., Goble, C., Grethe, J. S., Heringa, J., `t Hoen, P. A. C., Hooft, R., Kuhn, T., Kok, R., Kok, J., Lusher, S. J., Martone, M. E., Mons, A., Packer, A. L., Persson, B., Rocca-Serra, P., Roos, M., van Schaik, R., Sansone S.-A., Schultes, E., Sengstag, T. Slater, T., Strawn, G., Swertz, M. A., Thompson, M., van der Lei, J., van Mulligen, E., Veltrerop, J. Waangmeester, A., Wittenburg, P., Wolstencroft, K., Zhao, J., and Mons, B.: The FAIR Guiding Principles for scientific data management and stewardship, Sci. Data, 3, 160018, https://doi.org/10.1038/sdata.2016.18, 2016.
Woda, J., Wen, T., Lemon, J., Marcon, V., Keeports, C. M., Zelt, F., Steffy, L. Y., and Brantley, S. L.: Methane concentrations in streams reveal gas leak discharges in regions of oil, gas, and coal development, Sci. Total Environ., 737, 140105, https://doi.org/10.1016/j.scitotenv.2020.140105, 2020.
Wu, Q.: whitebox: “WhiteboxTools”, R Frontend, v1.2.0, GitHub [code], https://github.com/giswqs/whiteboxR (last access: 14 June 2023), 2020.
Yang, L., Li, X., Yan, W., Ma, P., and Wang, J.: CH4 concentrations and emissions from three rivers in the Chaohu Lake watershed in southeast China, J. Integr. Agr., 11, 665–673, https://doi.org/10.1016/S2095-3119(12)60054-9, 2012.
Yang, X., Pavelsky, T. M., Allen, G. H.: The past and future of global river ice, Nature, 577, 69–73, https://doi.org/10.1038/s41586-019-1848-1, 2020.
Zhang, L., Xia, X., Liu, S., Zhang, S., Li, S., Wang, J., Wang, G., Gao, H., Zhang, Z., Wang, Q., Wen, W., Liu, R., Yang, Z., Stanley, E. H., and Raymond, P. A.: Significant methane ebullition from alpine permafrost rivers on the East Qinghai–Tibet Plateau, Nat. Geosci., 13, 349–354, https://doi.org/10.1038/s41561-020-0571-8, 2020.
Zhang, W., Li, H., Pueppke, S. G., and Pang, J.: Restored riverine wetlands in a headwater stream can simultaneously behave as sinks of N2O and hotspots of CH4 production, Environ. Pollut., 284, 117114, https://doi.org/10.1016/j.envpol.2021.117114, 2021.
- Abstract
- Introduction
- Database components and assembly
- Results
- Discussion
- Code and data availability
- Conclusion
- Appendix A: GRiMeDB tables and variables
- Appendix B: Citations for data sources in GRiMeDB, including citations for unpublished data sets. Dates for unpublished data sets correspond to the year the data were provided by the data authors.
- Author contributions
- Competing interests
- Disclaimer
- Acknowledgements
- Financial support
- Review statement
- References
- Supplement
- Abstract
- Introduction
- Database components and assembly
- Results
- Discussion
- Code and data availability
- Conclusion
- Appendix A: GRiMeDB tables and variables
- Appendix B: Citations for data sources in GRiMeDB, including citations for unpublished data sets. Dates for unpublished data sets correspond to the year the data were provided by the data authors.
- Author contributions
- Competing interests
- Disclaimer
- Acknowledgements
- Financial support
- Review statement
- References
- Supplement