the Creative Commons Attribution 4.0 License.
the Creative Commons Attribution 4.0 License.
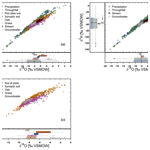
Integrated ecohydrological hydrometric and stable water isotope data of a drought-sensitive mixed land use lowland catchment
Doerthe Tetzlaff
Aaron Smith
Lukas Kleine
Hauke Daempfling
Jonas Freymueller
Chris Soulsby
Data from long-term experimental catchments are the foundation of hydrological sciences and are crucial for benchmarking process understanding, observing trends and natural cycles, and being prerequisites for testing predictive models. Integrated data sets which capture all compartments of our landscapes are particularly important in times of land use and climate change. Here, we present ecohydrological data measured at multiple spatial scales which allow differentiation of “blue” water fluxes (which maintain streamflow generation and groundwater recharge) and “green” water fluxes (which sustain vegetation growth). There are two particular unique aspects to this data set: (a) we measured water stable isotopes in the different landscape compartments (i.e. in precipitation, surface water, soil, groundwater, and plant water), and (b) we conducted this monitoring during the extreme drought of 2018 in central Europe. Stable water isotopes are so useful in hydrology as they provide “fingerprints” of the pathways water took when moving through a catchment. Thus, isotopes allow one to evaluate the dynamic relationships between water storage changes and fluxes, which is fundamental to understanding how catchments respond to hydroclimate perturbations or abrupt land use conversion. Second, as we provide the data until 2020, one can also investigate recovery of water stores and fluxes after extreme droughts. Last but not least, lowland headwaters are often understudied systems despite them providing important ecosystem services such as groundwater and drinking water provision and management for forestry and agriculture. The data are available at https://doi.org/10.18728/igb-fred-826.3 (Dämpfling, 2023).
- Article
(2757 KB) - Full-text XML
- BibTeX
- EndNote
Progress in scientific hydrology and provision of an evidence base for sustainable land and water management are only possible due to detailed, long-term observational data collected from experimental watersheds (Hewlett et al., 1969; Robinson et al., 2013). Such experimental “outdoor laboratories” are invaluable scientific resources given the complexity of increasing pressures on water supplies (e.g. Cosgrove and Loucks, 2015), land use change (Neill et al., 2021), and the uncertain effects and non-stationarity of projected climate change (Milly et al., 2015).
Ecohydrology adopts an interdisciplinary approach to investigate links between the structure and function of ecological systems and the partitioning, flux, and storage of freshwater (Guswa et al., 2020). Recent advances in monitoring and modelling have created manifold opportunities to address urgent ecohydrological questions on the importance of links between processes across the critical zone (CZ) – the dynamic, life-sustaining near surface of the terrestrial earth that extends between the tops of vegetation canopies, through the soil, and into groundwater (Grant and Dietrich, 2017). Within the CZ concept, vegetation plays a central and dynamic role in partitioning incoming precipitation into “blue-water” fluxes (streamflow generation and groundwater recharge) and “green-water” fluxes which maintain vegetation growth (Evaristo et al., 2015).
To enhance ecohydrological process understanding in catchment systems, robust, multi-scale integrated data sets are required (Tetzlaff et al., 2021). In this regard, water stable isotopes and other tracers can help identify sources and pathways of water in the landscape and across the CZ to elucidate how different land use affects water partitioning between green- and blue-water fluxes (Dubbert and Werner, 2019; Tetzlaff et al., 2015). Importantly, water stable isotopes have enhanced the characterization of the celerity of hydrological fluxes in different CZ compartments and quantification of the velocity of water particles and the associated mixing relationships in the sub-surface (Benettin et al., 2015; Birkel et al., 2011). Evaluating the dynamic relationships between water storage changes and fluxes is fundamental to understanding how catchments respond to hydroclimate perturbations, such as anomalous dry or wet periods or abrupt land use conversion. This provides a more nuanced and integrated understanding of how key ecohydrological couplings may be at risk during long-term changes in blue- and green-water partitioning resulting from climate and land use change (Orth and Destouni, 2018). Such integrated understanding is important in the context of projected increases in air temperature and aridity and in precipitation patterns, which may cause more variability in water availability, threatening the sustainability of important ecosystem services (Okruszko et al., 2011). As an increase in drought frequency and severity is expected across Europe as the 21st century progresses, the development of effective and evidence-based amelioration measures to underpin sustainable and integrated land and water management policies for changing climatic conditions is urgently needed (Samaniego et al., 2018).
Consequently, integrated ecohydrological and stable isotope data sets targeted at understanding the effects of different types of environmental change have outstanding potential, not least because interdisciplinary environmental research tends to give unanticipated insights (Burt, 1994). Such integrated data streams allow identification and quantification of the linkages between rainfall, soil moisture, groundwater, and runoff generation, facilitating deeper understanding of flood and drought risk in different types of landscapes and under different land use management (Huntingford et al., 2014).
Water resources in the extensive, glacially formed, lowland landscape of northern Europe, including the North German Plain, sustain food production (Gutzler et al., 2015; Barkmann et al., 2017) and water supplies to large cities like Berlin. Interestingly, such lowland catchments are still relatively understudied compared to more upland headwater landscapes with stronger topographic controls on drainage of surface and sub-surface water (Devito et al., 2005). In low-elevation catchments across the North German Plain, streams are usually groundwater-dominated, but the temporal and spatial heterogeneities in the hydrological functioning of these catchments are still not fully understood (Boulton and Hancock, 2006). For example, there is still a limited evidence base for quantifying how drought affects groundwater recharge and streamflow generation in lowland areas in central Europe, including the cessation of flow during the summer (Germer et al., 2011).
To help address these knowledge gaps, here, we present a comprehensive set of ecohydrological hydrometric and stable water isotope data of 2 years for the Demnitzer Mill Creek catchment, north-eastern Germany. The data set is unique in its integrative characteristics that the different compartments of the CZ were sampled across a mesoscale catchment in terms of their isotopic signature and supporting ecohydrological data. By coincidence, these first 2 years, of what will be a long-term study, captured the changing impacts of a prolonged drought period (2018–2020) with a strong negative rainfall anomaly that became the most severe regional drought so far in the 21st century (Kleine et al., 2021a). The data allow the effects of droughts (and their persistence) on water storage, fluxes, and age dynamics in the CZ to be investigated (Smith et al., 2022). Our objective here is to provide this high-spatiotemporal-resolution ecohydrological data set to improve understanding of the storages and flow pathways of both blue and green water across processes at the larger catchment scale in lowland catchments. We are continuing these observations to assess long-term climatic trends in this drought-sensitive region of north-eastern Germany, which is characterized by high water losses due to evapotranspiration and poor water retention in the widespread sandy soils (Smith et al., 2021). Further, these data can potentially be used to understand the hydrologic functioning of other drought-sensitive regions beyond north-eastern Germany.
The data presented here were monitored in the Demnitzer Mill Creek catchment (DMC) located in north-eastern Germany (52∘23′ N, 14∘15′ E; Fig. 1, Table 1). The DMC is a lowland drought-sensitive area south-east of Berlin, the German capital, and situated in the North German Plain. The region has high socioeconomic significance through the provision of numerous ecosystem services, including food security, timber production, groundwater recharge, and river flow generation, which sustain drinking water supplies for Berlin (Kleine et al., 2021a). The original motivation behind establishing DMC as an observatory in 1990 was to investigate the impact of agricultural pollutants on surface water quality (Gelbrecht et al., 2005).
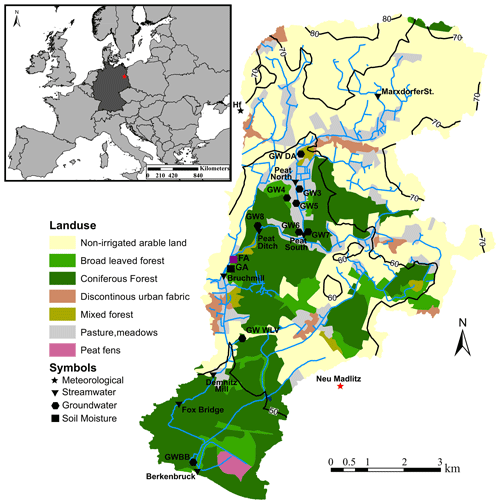
Figure 1The Demnitzer Mill Creek catchment and its location within Europe and Germany. Measurement types are indicated in the legend, with red indicating no isotope measurements, black and purple indicating isotope measurements, and purple additionally indicating sap flow and xylem isotope measurements. Meteorological measurements at Neu Madlitz were conducted by the German Weather Service (DWD – Deutscher Wetterdienst).
Table 1Overview of the properties of the Demnitzer Mill Creek catchment at the catchment outlet. The overview includes physiological characteristics, land use, and geology.
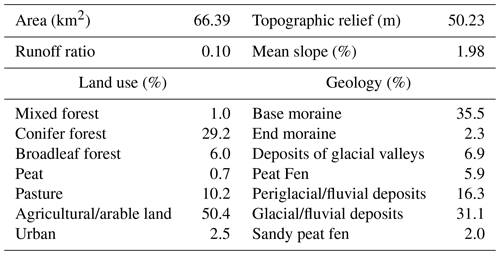
The hydroclimate is temperate, with warm, humid summers (Kottek et al., 2006). Mean annual precipitation and air temperature are 567 mm yr−1 and 9.6 ∘C, respectively (DWD, 2020, for 2006–2015). Seasonal contrasts are characterized by higher summer precipitation, mainly from high-intensity convective events, and slightly lower precipitation during frequent frontal-rainfall events in winter. The landscape was shaped by the last glaciation (Weichselian); soils are predominantly sandy and formed on glacial and fluvial deposits (Kleine et al., 2021b). The catchment is dominated by groundwater and likely had little surface runoff before human intervention. Previously, numerous peat fens and freshwater lakes in hollows existed, but these were drained during a long history of anthropogenic management (Nützmann et al., 2011). Land use is currently dominated by farming and forestry (Kleine et al., 2020; Smith et al., 2020b). The catchment is also relatively sparsely populated and has recently experienced recolonization of beaver (Smith et al., 2020a) and wolf and even sporadic sighting of elk.
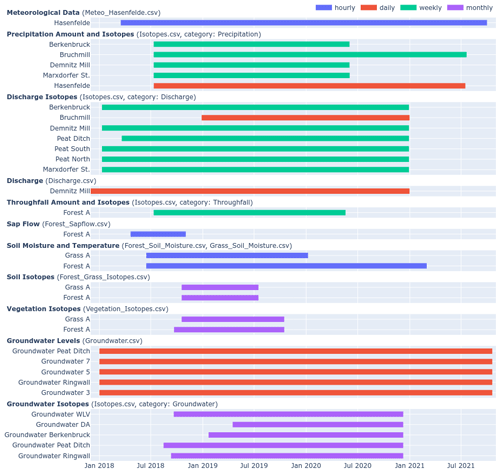
Figure 2Measurement period and temporal resolution (colour code) for each parameter at each site within the Demnitzer Mill Creek catchment, including meteorological, soil, vegetation, stream, and groundwater hydrological and isotope data sets.
Maintenance of crucial ecosystem services in the landscape is dependent on sufficient seasonal precipitation input to sustain adequate soil moisture levels in the rooting zone to support crop and tree growth (Drastig et al., 2011) and acceptable groundwater recharge to sustain groundwater–surface water exchanges. However, high water losses due to evapotranspiration (∼90 % of total precipitation), particularly from forested areas, and poor water retention in the widespread sandy soils (Smith et al., 2021) result in catchment drought sensitivity (Kleine et al., 2020). Further, increased flow disconnections and fragmentation of the stream network occur during droughts (Kleine et al., 2021a; Smith et al., 2021).
3.1 Instrumentation overview
A fully automatic weather station (AWS) was installed and has been operated in Hasenfelde (Hf, Fig. 1, Table 2) since April 2018, including net radiation, air temperature, relative humidity, precipitation, and ground heat flux every 15 min. Weekly cumulative precipitation was additionally collected at four locations nested from north to south in the catchment, Marxdorfer St., Demnitz Mill, Bruchmill, and Berkenbruck (Figs. 1 and 2, Table 2), from July 2018 to April 2020. Throughfall was collected under the canopy at Forest A at five locations (Forest A1–5) within a 10 m square fenced area. Throughfall was collected using standard rain gauges (Rain gauge kit, S. Brannan & Sons, Cleator Moor, UK).
Table 2Overview of the site locations in DMC, including the site name, coordinates, data collected, start and end dates, and resolution. P is precipitation, GW is groundwater level, THR is throughfall, Ts is soil temperature, va is wind speed/direction, Ta is air temperature, Pa is air pressure, RH is relative humidity, NR is net radiation, and Sap is sap flow. Veg is vegetation samples. Subscript “iso” indicates isotope samples.
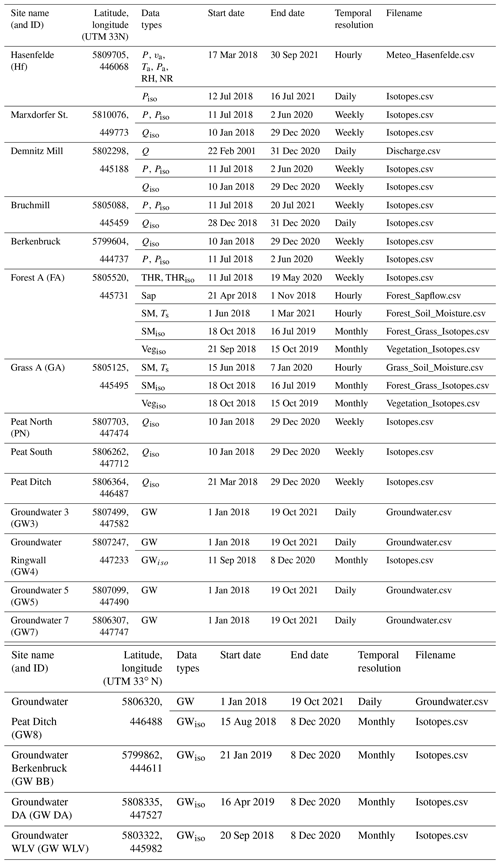
Soil moisture and temperature profiles were established at Forest A (FA) and Grass A (GA) in June 2018 with 18 sensors per site (SMT-100, Umwelt-Geräte-Technik GmbH, Müncheberg, Germany, Table 2). The sensors were distributed equally at soil depths of 20, 60, and 100 cm at each site (i.e. three sensors per depth), measuring every 15 min with a precision of ±3 % for volumetric soil water content and ±0.2 ∘C for soil temperature.
Sap-flow measurements (Table 2) were established in 12 trees at Forest A, including Scots Pine (Pinus sylvestris), European Oak (Quercus robur), common hazel (Corylus avellana), and Red Oak (Quercus rubra). Measurements were conducted using two to four radially installed thermal-dissipation-based sap-flow sensors (TDP probes, Dynamix Inc., Houston, TX, USA, precision 0.001 ∘C). Sap-flow measurements were recorded every 15 min. Sensors were installed at approximately 1.3 m above the ground. The tree diameter was also measured at this height (DBH; mean: 76 cm; SD: 35 cm). All sensors consisted of two thermometers installed in the sapwood at 4 cm vertical distance from each other and were shielded from external sources of temperature change (e.g. radiation). The upper thermometer was heated, and differences in temperature were collected hourly with a CR1000 data logger (Campbell Scientific, USA). The temperature difference was used to calculate flux velocity and was combined with the sapwood area to calculate a flux rate. Conditions of zero transpiration were determined from daily maximum temperature differences. The resulting flux rate per unit of sapwood area was adjusted to the plot using a ratio of sapwood area to forest area that was established with 10 trees. More details can be found in Kleine et al. (2020).
Streamwater level was measured at Demnitz Mill (beginning in 1986). Water-level measurements were established by the Leibniz Institute of Freshwater Ecology and Inland Fisheries and recorded with divers (Micro 10 m and Baro divers, Van Essen Instruments; accuracy ±0.5 cm). The diver set-up includes an internal atmospheric pressure correction. Water level has also been recorded since 1982 at Berkenbruck using pressure transducers and is collected by the Landesamt für Umwelt, Brandenburg (all discharge data can be acquired there). Channel stability at Demnitz Mill has permitted rating-curve development to translate water-level measurements to discharge at this location (which is provided in the data sheet).
Groundwater-level divers were installed at five locations throughout the catchment in 2001 (GW3, GW4, GW5, GW7, and GW8) (Figs. 1 and 2). The groundwater level at each site was measured every 4 h with an AquiLite ATP-10 diver (accuracy > 0.1 %; AquiTronic Umweltmeßtechnik GmbH, Kirchheim/Teck, Germany) with internal correction for atmospheric pressure.
3.2 Isotope sampling overview
A modified autosampler (ISCO 3700, Teledyne Isco, Lincoln, USA) was installed nearby the AWS Hasenfelde to collect daily samples of precipitation for water stable isotope analysis (all isotope samples are listed in Table 2 with the subscript “iso”). Daily streamwater samples for stable water isotope analysis were collected at Bruchmill from an autosampler (ISCO 3700, Teledyne Isco, Lincoln, USA), which was established in December 2018. Manual sampling from different locations and different water cycle/landscape compartments supplemented the autosamplers. Samples were taken from the weekly cumulative precipitation at four locations (Marxdorfer Str, Bruchmill, Demnitz Mill, Berkenbruck) and throughfall (Forest A, five locations there) (Fig. 2). Further, monthly samples of soil water isotopes were taken at six depths (2.5, 7.5, 15, 30, 60, 90 cm) in triplicate for Forest A and Grass A. This was complemented by synoptic, spatially distributed sampling of the upper 30 cm in 2019. Samples were placed in a sterile zip-lock bag (CB400-420siZ, Weber Packaging GmbH, Güglingen, Germany) and analysed using the direct water vapour equilibrium method (Wassenaar et al., 2008). Weekly grab samples of streamwater were taken at all nested streamwater locations (eight locations; Fig. 1). Groundwater isotopes were sampled at five groundwater wells (GW4, GW8, GW DA, GW BB, GW WLV). Vegetation isotopic sampling was conducted by taking twig samples from different vegetation in Forest A and samples of the non-green stem of the grass at site Grass A. Vegetation samples were stored at −20 ∘C after sampling until analysis.
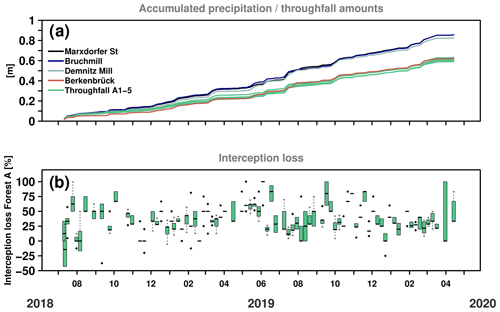
Figure 3(a) Cumulative precipitation and throughfall at multiple (nested) locations throughout the catchment. Throughfall was collected weekly at Forest A with (b) five samplers (1–5) distributed throughout a 10 m square fenced area. Open-area precipitation at Bruchmill (nearby Forest A) was used to calculate weekly interception loss.
A layer of paraffin was added to the bottom of all autosampler containers to prevent evaporation and fractionation from collected water. Autosamplers are emptied each week. Collected weekly precipitation, throughfall, streamwater, and groundwater were sealed and refrigerated until isotopic analysis (usually within 1 week).
All liquid water samples (isotopes in precipitation Piso, in throughfall THRiso, in streamwater Qiso, and in groundwater GWiso) were filtered (0.2 µm, cellulose acetate, Lab Logistics Group GmbH, Meckenheim, Germany) and cooled before being analysed using cavity ring-down spectroscopy (CRDS, L2130-i, Picarro, Inc., CA, USA). Additionally, the CRDS was used for the analysis of soil water extracted via the direct liquid water equilibrium method. Vegetation samples were extracted in January 2020 using the cryogenic extraction method given in Dubbert et al. (2013) and analysed with the CRDS. For all CRDS analyses, we used four standards for a linear correction function and standards of the International Atomic Energy Agency (IAEA) for calibration. After quality-checking and averaging multiple analyses for each sample, the results were expressed in δ notation with Vienna Standard Mean Ocean Water (VSMOW). Analytical precision was 0.05 ‰ standard deviation (SD) for δ18O and 0.14 ‰ SD for δD. To screen for interference from organics, the ChemCorrect software (Picarro, Inc.) was applied and contaminated samples discarded. Liquid samples were injected six times and the first three injections discarded. As an index for instrument uncertainty, standard deviations of all isotope samples are given in the accompanying data sheet.
Monitoring for precipitation commenced in the 2018 summer drought when low rainfall inputs continued through the following winter (Fig. 3a). Large rainfall events (>20 mm d−1) were relatively rare and mostly summer convectional storms. Even by summer 2020, most months had below-average rainfall. Throughfall at the Forest A site typically was 70 %–90 % of incident rainfall (measured as open precipitation at Bruchmill nearby), with higher interception losses in low-intensity summer storms and the lowest in winter or high-intensity summer storms. Heterogeneity in throughfall was marked (Fig. 3b), emphasizing the importance of the forest canopy in redistributing net rainfall to the forest floor.
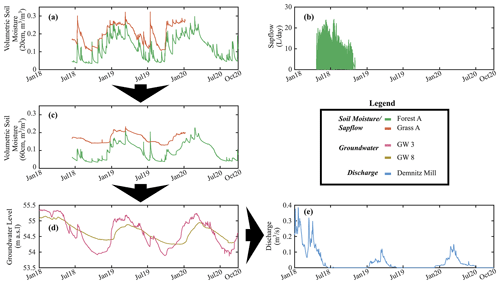
Figure 4(a) Shallow soil moisture, (b) sap flow, (c) deep soil moisture, (d) groundwater levels from two wells, and (e) discharge from Demnitz Mill Creek station. Arrows show connections between layers and fluxes. * Groundwater 3 is within the wetland and Groundwater 8 is outside the wetland (near the areas of Forest A and Grass A; see Fig. 1).
Rainfall fluxes mostly drove short-term soil moisture variations (Fig. 4a and c), which were more responsive in the upper soil layers (at 20 cm) than deeper layers. There was higher variability in volumetric soil moisture under forested land cover, where soils are sandier and more structured and effective rainfall is lower due to interception losses. Seasonality in evapotranspiration (usefully indexed by sap flow in Fig. 4b) modulated the effects of rainfall on soil moisture storage. Seasonal soil moisture dynamics also governed groundwater recharge and variation in groundwater levels, which had an annual range of ∼1.5 m at well G3 and ∼1 m at the Peat Ditch well (Fig. 4d). Despite clear winter recharge and spring drawdown in each well, peak winter and summer levels were lower in 2019 and still in 2020 despite a slight recovery compared to 2018, indicating the cumulative “memory effects” of the drought. This was also evident in the stream hydrograph with very low discharge peaks in 2019 and 2020, which also had prolonged periods where flow ceased in the summer. Thus, winter soil moisture replenishment was insufficient to match long-term groundwater recharge. These different correlations underline the added value of simultaneous data from long-term study sites on transpiration, soil water, groundwater, and streamflow as droughts develop (Smith et al., 2022).
Stable water isotope signatures in precipitation showed high day-to-day variability superimposed on strong seasonality, with more depleted values in winter and more enriched values in summer (Fig. 5a). Interestingly, weekly throughfall signatures were very similar to the (weekly and daily) precipitation signal, showing no strong signs of evaporative fractionation during canopy storage (Fig. 5b). This likely reflects the high-intensity nature of most summer rainfall, which affords limited opportunity for canopy evaporation. Streamwater signatures at all nested sites showed similar seasonality but much more damping in the signal (Fig. 5c). Groundwater was the most damped and similar in composition to streamflow during winter (Fig. 5d). In summer, sites downstream of Marxdorfer Str showed evidence of evaporative fractionation from either the channel network or riparian soils and plotted below the meteoric water line before streamflow ceased. Monthly soil water samples showed higher variability in isotopic composition under forest than under grass, mainly reflecting soil characteristics with more retentive, loamy, and wetter soils at the grassland site buffering the effects of rainfall inputs. At both vegetation sites, seasonal variation in isotopic composition tracked precipitation, though in deeper soil, the isotopic signal was more damped. Vegetation samples from the oaks showed higher variation than from grass.
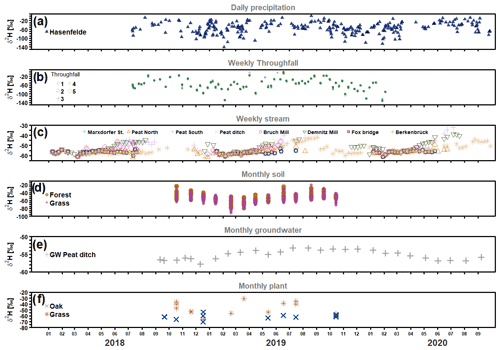
Figure 5Time series of deuterium (δ2H) in (a) daily precipitation at Hasenfelde, (b) weekly throughfall (in Forest A), (c) streamwater (daily at Bruchmill and weekly at the other sampling locations), (d) soil water under forest and grass, (e) groundwater at well GW8/Peat Ditch, and (f) plant samples at various locations (from oaks in Forest A and grass in Grass A) in the catchment.
Differences in the isotope dynamics of different critical-zone compartments are shown in the dual-isotope space in Fig. 6a. The damping of precipitation in groundwater and streamflow is apparent, as is the fractionation of more enriched summer streamflow samples (Fig. 6b). The role of the soil in partitioning water is apparent from the overlap between deeper soil horizons and groundwater, which were both more weighted to winter precipitation – when recharge is greatest (Fig. 6c). Xylem water in oaks and grass (measured at Forest A) tended to show the effects of fractionation, which was most marked in the oaks and may point to different soil water sources of root uptake.
All data presented in this paper are available from the IGB open-data repository FRED (https://doi.org/10.18728/igb-fred-826.3; Dämpfling, 2023) under a Creative Commons Attribution 4.0 International Public License (CC BY 4.0) including detailed metadata and contact information for any further questions.
The integrated data set presented in this paper is unique because (1) it captures complicated ecohydrological dynamics over 2 years during an exceptional drought (in 2018/2019) in central Europe, (2) the different compartments of the critical zone were monitored through stable water isotope data and complementary ecohydrological data for contrasting land use, and (3) multi-scale, nested catchment time series were derived. In total, 11 CSV files with over 100 000 rows of data from 18 different geographic locations are available. The data are quality-controlled. We included meteorological data and precipitation and throughfall amount. Catchment response data include stream discharge at the catchment outlet and another nested site and stream-level data at two further sites, soil moisture from multiple depths at two locations (two different land uses), groundwater-level data at five locations, and sap-flow measurements from one forest location. Stable water isotope data include precipitation water, throughfall, streamwater at eight sites, soil water isotopes from two sites plus spatially distributed samples of upper soils, vegetation samples at two locations, and groundwater at six locations. Data continue to be collected, and updated data sets will be published based on available resources.
As such, these data provide an excellent, integrated ecohydrological perspective on the drought response of a lowland agricultural landscape. Such data are of course important in their own right but are equally invaluable for challenging environmental models as constraints on internal model function that can be used to increase confidence in the use of models in projecting the impacts of future change. Integrated data like the ones summarized here are also important for a range of scientific questions that are growing in importance as the effects of climate change become more apparent. These include understanding how droughts develop and propagate through components of hydrological systems and compartments of the critical zone. What are the effects of land cover on this propagation and how does it affect water cycling in vegetation? How long does recovery of different system components take once rainfall anomalies become positive? How resilient are different critical-zone compartments or entire landscapes against climate extremes such as droughts? Hopefully, this data set will be used by scientists to increase understanding of critical issues such as what the water footprints of alternative land uses are and how these can be reduced whilst maintaining societal needs. This will help to contribute to the development of more sustainable and resilient land and water management policies that will be needed in the face of increased longevity and frequency of droughts.
AS, HD, and LK prepared the data sets. Data sets were collected by LK and JF. DT, CS, and AS prepared the manuscript with contributions from all the co-authors.
The contact author has declared that none of the authors has any competing interests.
Any reference to specific equipment types or manufacturers is for informational purposes and does not represent product endorsement. IGB is an equal-opportunity provider.
Publisher's note: Copernicus Publications remains neutral with regard to jurisdictional claims in published maps and institutional affiliations.
We thank David Dubbert for support of the liquid water isotope samples. We also thank our colleagues from the Finck Foundation, Benedict Boesel and Max Kuester, for the trustful collaboration and for providing access to the study sites.
We were funded by the Bundesministerium für Bildung und Forschung (BMBF – funding code 033W034A), which supported the stable isotope laboratory at IGB. Funding for Doerthe Tetzlaff was also received through the Einstein Research Unit “Climate and Water under Change” from the Einstein Foundation Berlin and Berlin University Alliance (grant no. ERU-2020-609).
This paper was edited by Conrad Jackisch and reviewed by two anonymous referees.
Barkmann, T., Siebert, R., and Lange, A.: Land-use experts' perception of regional climate change: An empirical analysis from the North German Plain, Climatic Change, 144, 287–301, https://doi.org/10.1007/s10584-017-2041-x, 2017.
Benettin, P., Bailey, S. W., Campbell, J. L., Green, M. B., Rinaldo, A., Likens, G. E., McGuire, K. J., and Botter, G.: Linking water age and solute dynamics in streamflow at the Hubbard Brook Experimental Forest, NH, USA, Water Resour. Res., 51, 9256–9272, https://doi.org/10.1002/2015WR017552, 2015.
Birkel, C., Tetzlaff, D., Dunn, S. M., and Soulsby, C.: Using lumped conceptual rainfall–runoff models to simulate daily isotope variability with fractionation in a nested mesoscale catchment, Adv. Water Resour., 34, 383–394, https://doi.org/10.1016/j.advwatres.2010.12.006, 2011.
Boulton, A. J. and Hancock, P. J.: Rivers as groundwater-dependent ecosystems: A review of degrees of dependency, riverine processes and management implications, Aust. J. Bot., 54, 133–144, https://doi.org/10.1071/BT05074, 2006.
Burt, T. P.: Long-term study of the natural environment – Perceptive science or mindless monitoring?, Prog. Phys. Geogr., 18, 475–496, https://doi.org/10.1177/030913339401800401, 1994.
Cosgrove, W. J. and Loucks, D. P.: Water management: Current and future challenges and research directions, Water Resour. Res., 51, 4823–4839, https://doi.org/10.1002/2014WR016869, 2015.
Dämpfling, H.: Demnitz Isotope Hydrological Data, IGB Leibniz-Institute of Freshwater Ecology and Inland Fisheries, FRED [data set], https://doi.org/10.18728/igb-fred-826.3, 2023.
Devito, K., Creed, I., Gan, T., Mendoza, C., Petrone, R., Silins, U., and Smerdon, B.: A framework for broad-scale classification of hydrologic response units on the Boreal Plain: Is topography the last thing to consider?, Hydrol. Process., 19, 1705–1714, https://doi.org/10.1002/hyp.5881, 2005.
Drastig, K., Prochnow, A., Baumecker, M., Berg, W., and Brunsch, R.: Agricultural Water Management in Brandenburg, J. Geogr. Soc. Berlin, 142, 119–140, 2011.
Dubbert, M. and Werner, C.: Water fluxes mediated by vegetation: Emerging isotopic insights at the soil and atmosphere interfaces, New Phytol., 221, 1754–1763, https://doi.org/10.1111/nph.15547, 2019.
Dubbert, M., Cuntz, M., Piayda, A., Maguás, C., and Werner, C.: Partitioning evapotranspiration – Testing the Craig and Gordon model with field measurements of oxygen isotope ratios of evaporative fluxes, J. Hydrol., 496, 142–153, https://doi.org/10.1016/j.jhydrol.2013.05.033, 2013.
DWD – Deutscher Wetterdienst: Climate Data Center (CDC), https://cdc.dwd.de/portal/ (last access: 4 April 2023), 2020.
Evaristo, J., Jasechko, S., and McDonnell, J. J.: Global separation of plant transpiration from groundwater and streamflow, Nature, 525, 91–94, https://doi.org/10.1038/nature14983, 2015.
Gelbrecht, J., Lengsfeld, H., Pöthig, R., and Opitz, D.: Temporal and spatial variation of phosphorus input, retention and loss in a small catchment of NE Germany, J. Hydrol., 304, 151–165, https://doi.org/10.1016/j.jhydrol.2004.07.028, 2005.
Germer, S., Kaiser, K., Bens, O., and Hüttl, R. F.: Water Balance Changes and Responses of Ecosystems and Society in the Berlin-Brandenburg Region – a Review, J. Geogr. Soc. Berlin, 142, 65–95, 2011.
Grant, G. E. and Dietrich, W. E.: The frontier beneath our feet, Water Resour. Res., 53, 2605–2609, https://doi.org/10.1002/2017WR020835, 2017.
Guswa, A. J., Tetzlaff, D., Selker, J. S., Carlyle-Moses, D. E., Boyer, E. W., Bruen, M., Cayuela, C., Creed, I. F., van de Giesen, N., Grasso, D., Hannah, D. M., Hudson, J. E., Hudson, S. A., Iida, S., Jackson, R. B., Katul, G. G., Kumagai, T., Llorens, P., Lopes Ribeiro, F., Michalzik, B., Nanko, K., Oster, C., Pataki, D. E., Peters, C. A., Rinaldo, A., Sanchez Carretero, D., Trifunovic, B., Zalewski, M., and Levia, D. F.: Advancing ecohydrology in the 21st century: A convergence of opportunities, Ecohydrology, 13, e2208, https://doi.org/10.1002/eco.2208, 2020.
Gutzler, C., Helming, K., Balla, D., Dannowski, R., Deumlich, D., Glemnitz, M., Knierim, A., Mirschel, W., Nendel, C., Paul, C., Sieber, S., Stachow, U., Starick, A., Wieland, R., Wurbs, A., and Zander, P.: Agricultural land use changes – a scenario-based sustainability impact assessment for Brandenburg, Germany, Ecol. Indic., 48, 505–517, https://doi.org/10.1016/j.ecolind.2014.09.004, 2015.
Hewlett, J. D., Lull, H. W., and Reinhart, K. G.: In Defense of Experimental Watersheds, Water Resour. Res., 5, 306–316, https://doi.org/10.1029/WR005i001p00306, 1969.
Huntingford, C., Marsh, T., Scaife, A. A., Kendon, E. J., Hannaford, J., Kay, A. L., Lockwood, M., Prudhomme, C., Reynard, N. S., Parry, S., Lowe, J. A., Screen, J. A., Ward, H. C., Roberts, M., Stott, P. A., Bell, V. A., Bailey, M., Jenkins, A., Legg, T., Otto, F. E. L., Massey, N., Schaller, N., Slingo, J., and Allen, M. R.: : Potential influences on the United Kingdom's floods of winter 2013/14, Nat. Clim. Change, 4, 769–777, https://doi.org/10.1038/nclimate2314, 2014.
Kleine, L., Tetzlaff, D., Smith, A., Wang, H., and Soulsby, C.: Using water stable isotopes to understand evaporation, moisture stress, and re-wetting in catchment forest and grassland soils of the summer drought of 2018, Hydrol. Earth Syst. Sci., 24, 3737–3752, https://doi.org/10.5194/hess-24-3737-2020, 2020.
Kleine, L., Tetzlaff, D., Smith, A., Goldhammer, T., and Soulsby, C.: Using isotopes to understand landscape-scale connectivity in a groundwater-dominated, lowland catchment under drought conditions, Hydrol. Process., 35, e14197, https://doi.org/10.1002/hyp.14197, 2021a.
Kleine, L., Tetzlaff, D., Smith, A., Dubbert, M., and Soulsby, C.: Modelling ecohydrological feedbacks in forest and grassland plots under a prolonged drought anomaly in central Europe 2018–2020, Hydrol. Process., 35, e14325, https://doi.org/10.1002/hyp.14325, 2021b.
Kottek, M., Grieser, J., Beck, C., Rudolf, B., and Rubel, F.: World Map of the Köppen–Geiger Climate Classification Updated, Meteorol. Z., 15, 259–263, https://doi.org/10.1127/0941-2948/2006/0130, 2006.
Milly, P. C. D., Betancourt, J., Falkenmark, M., Hirsch, R. M., Kundzewicz, Z. W., Lettenmaier, D. P., Stouffer, R. J., Dettinger, M. D., and Krysanova, V.: On Critiques of “Stationarity is Dead: Whither Water Management?”, Water Resour. Res., 51, 7785–7789, https://doi.org/10.1002/2015WR017408, 2015.
Neill, A. J., Birkel, C., Maneta, M. P., Tetzlaff, D., and Soulsby, C.: Structural changes to forests during regeneration affect water flux partitioning, water ages and hydrological connectivity: Insights from tracer-aided ecohydrological modelling, Hydrol. Earth Syst. Sci., 25, 4861–4886, https://doi.org/10.5194/hess-25-4861-2021, 2021.
Nützmann, G., Wolter, C., Venohr, M., and Pusch, M.: Historical Patterns of Anthropogenic Impacts on Freshwaters in the Berlin-Brandenburg Region, J. Geogr. Soc. Berlin, 142, 41–64, 2011.
Okruszko, T., Duel, H., Acreman, M., Grygoruk, M., Flörke, M., and Schneider, C.: Broad-scale ecosystem services of European wetlands- – Overview of the current situation and future perspectives under different climate and water management scenarios, Hydrolog. Sci. J., 56, 1501–1517, https://doi.org/10.1080/02626667.2011.631188, 2011.
Orth, R. and Destouni, G.: Drought reduces blue-water fluxes more strongly than green-water fluxes in Europe, Nat. Commun., 9, 3602, https://doi.org/10.1038/s41467-018-06013-7, 2018.
Robinson, M., Rodda, J. C., and Sutcliffe, J. V.: Long-term environmental monitoring in the UK: Origins and achievements of the Plynlimon catchment study, Trans. Inst. Brit. Geogr., 38, 451–463, 2013.
Samaniego, L., Thober, S., Kumar, R., Wanders, N., Rakovec, O., Pan, M., Zink, M., Sheffield, J., Wood, E. F., and Marx, A.: Anthropogenic warming exacerbates European soil moisture droughts, Nat. Clim. Change, 8, 421–426, https://doi.org/10.1038/s41558-018-0138-5, 2018.
Smith, A., Tetzlaff, D., Gelbrecht, J., Kleine, L., and Soulsby, C.: Riparian wetland rehabilitation and beaver re-colonization impacts on hydrological processes and water quality in a lowland agricultural catchment, Sci. Total Environ., 699, 134302, https://doi.org/10.1016/j.scitotenv.2019.134302, 2020a.
Smith, A., Tetzlaff, D., Kleine, L., Maneta, M. P., and Soulsby, C.: Isotope-aided modelling of ecohydrologic fluxes and water ages under mixed land use in Central Europe: The 2018 drought and its recovery, Hydrol. Process., 34, 3406–3425, https://doi.org/10.1002/hyp.13838, 2020b.
Smith, A., Tetzlaff, D., Kleine, L., Maneta, M., and Soulsby, C.: Quantifying the effects of land use and model scale on water partitioning and water ages using tracer-aided ecohydrological models, Hydrol. Earth Syst. Sci., 25, 2239–2259, https://doi.org/10.5194/hess-25-2239-2021, 2021.
Smith, A., Tetzlaff, D., Maneta, M., and Soulsby, C.: Critical zone response times and water age relationships under variable catchment wetness states: insights using a tracer-aided ecohydrological model, Water Resour. Res., 48, e2021WR030584, https://doi.org/10.1029/2021WR030584, 2022.
Tetzlaff, D., Buttle, J., Carey, S. K., van Huijgevoort, M. H. J., Laudon, H., McNamara, J. P., Mitchell, C. P. J., Spence, C., Gabor, R. S., and Soulsby, C.: A preliminary assessment of water partitioning and ecohydrological coupling in northern headwaters using stable isotopes and conceptual runoff models, Hydrol. Process., 29, 5153–5173, https://doi.org/10.1002/hyp.10515, 2015.
Tetzlaff, D., Buttle, J., Carey, S. K., Kohn, M. J., Laudon, H., McNamara, J. P., Smith, A., Sprenger, M., and Soulsby, C.: Stable isotopes of water reveal differences in plant–soil water relationships across northern environments, Hydrol. Process., 35, e14023, https://doi.org/10.1002/hyp.14023, 2021.
Wassenaar, L. I., Hendry, M. J., Chostner, V. L., and Lis, G. P.: High resolution pore water δ2H and δ18O measurements by H2O(liquid)–H2O(vapor) equilibration laser spectroscopy, Environ. Sci. Technol., 42, 9262–9267, https://doi.org/10.1021/es802065s, 2008.