the Creative Commons Attribution 4.0 License.
the Creative Commons Attribution 4.0 License.
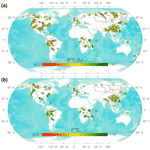
MOdern River archivEs of Particulate Organic Carbon: MOREPOC
Yutian Ke
Damien Calmels
Julien Bouchez
Cécile Quantin
Riverine transport of particulate organic carbon (POC) associated with terrigenous solids to the ocean has an important role in the global carbon cycle. To advance our understanding of the source, transport, and fate of fluvial POC from regional to global scales, databases of riverine POC are needed, including elemental and isotope composition data from contrasted river basins in terms of geomorphology, lithology, climate, and anthropogenic pressure. Here, we present a new, open-access, georeferenced, and global database called MOdern River archivEs of Particulate Organic Carbon (MOREPOC) version 1.1, featuring data on POC in suspended particulate matter (SPM) collected at 233 locations across 121 major river systems. This database includes 3546 SPM data entries, among them 3053 with POC content, 3402 with stable carbon isotope (δ13C) values, 2283 with radiocarbon activity (Δ14C) values, 1936 with total nitrogen content, and 299 with an aluminum-to-silicon ratio (Al Si). The MOREPOC database aims at being used by the Earth system community to build comprehensive and quantitative models for the mobilization, alteration, and fate of terrestrial POC. The database is made available on the Zenodo repository in machine-readable formats as a data table and GIS shapefile at https://doi.org/10.5281/zenodo.7055970 (Ke et al., 2022).
- Article
(9653 KB) - Full-text XML
- BibTeX
- EndNote
Rivers are the main conveyor of terrestrial material to the ocean in the form of suspended particulate matter (SPM), which carries particulate organic carbon (POC) (Leithold et al., 2016; Blair and Aller, 2012). POC is defined as the fraction of total organic carbon contained in the solid fraction recovered after filtration of river water. Before reaching the coastal environment and being eventually buried at the ocean bottom, terrestrial POC may experience alteration and/or degradation processes during fluvial transport. These processes need to be better quantified as they are key features of the global carbon cycle, particularly in the context of current global environmental changes.
Riverine POC is a mixture of organic carbon (OC or Corg) from various sources and can be split into two major origins: biospheric POC (POCbio) and petrogenic POC (POCpetro) (Blair et al., 2003, 2004; Galy et al., 2007; Hilton et al., 2008). Land plants, soils, aquatic organisms, and microbes can all contribute radiocarbon-active riverine POC, with ages ranging from modern to multi-millennial (Galy et al., 2007; Blair et al., 2010; Hilton et al., 2011). Radiocarbon-dead POCpetro is derived from the erosion of sedimentary rocks, consists of terrestrial or marine organic carbon photosynthesized millions of years ago, and has survived to at least one full erosion–sedimentation–exhumation cycle (Galy et al., 2008a; Hilton et al., 2011). The balance between the release of CO2 by oxidation of POCpetro and the drawdown of CO2 by burial of POCbio in marine sediments controls the impact of the OC cycle on atmospheric CO2 level over geological timescales (> 100 000 years). The resulting long-term global carbon fluxes are similar in magnitude to those from silicate weathering and volcanism (Berner, 2003; Hilton et al., 2014; Petsch, 2014; Galy et al., 2007; Galy and Eglinton, 2011; Hilton and West, 2020). Net continental POCbio burial accounts for about 35–70 Mt C yr−1 considering that only 30 % of the total riverine input to the ocean is efficiently buried (Blair and Aller, 2012; Burdige, 2005; Galy et al., 2015), while the oxidation of POCpetro in sedimentary rocks would release about 40–100 Mt C yr−1 into the atmosphere (Petsch, 2014; Hilton and West, 2020). These fluxes are comparable to those induced by silicate weathering, carbonate weathering by oxidation of sulfides, and volcanism, demonstrating that POC could play an important role in the Earth's long-term carbon cycle (Berner, 2003; Hilton et al., 2014; Petsch, 2014; Galy et al., 2007; Galy and Eglinton, 2011; Hilton and West, 2020). Consequently, it is fundamental for quantifying POC sources and fluxes as well as understanding the fate of the different POC pools, in order to better constrain the role that POC plays in the global carbon cycle. With that aim, radiocarbon activity provides unique information on POC age, residence time, and source. Thanks to improved carbon-dating technology and more easily accessible accelerator mass spectrometry (AMS, Wacker et al., 2010), routine and high-precision radiocarbon dating has been extensively applied for the analysis of radiocarbon abundance in riverine POC during the last 2 decades. Together with the stable isotope composition of the carbon (13C 12C ratio, expressed as δ13C), POC content, or other organic–inorganic proxies (e.g., organic carbon-to-nitrogen Corg N ratio, aluminum-to-organic carbon Al OC ratio), radiocarbon activity helps to constrain the source, transport, and fate of riverine POC (Raymond and Bauer, 2001).
Globally, rivers drain areas of contrasted lithology, climate, tectonics, vegetation, and anthropogenic pressure, parameters that can all impact riverine POC fluxes. At the global scale, riverine POCbio is known to be dominantly sourced from soil organic carbon (SOC) (e.g., Tao et al., 2015; Wu et al., 2018; Wild et al., 2019), whose turnover time (the ratio of OC stock to OC input flux in soil) and thus radiocarbon activity are greatly controlled by temperature and precipitation (Shi et al., 2020; Eglinton et al., 2021). In permafrost regions, SOC has a longer turnover time and is depleted in 14C, whereas SOC with the shortest turnover time and the most enriched 14C signature is found in tropical forests and savannahs (Shi et al., 2020; Carvalhais et al., 2014). Consequently, riverine POC is significantly older in Arctic rivers (e.g., Kolyma, Lena) than in tropical rivers such as the Congo or Amazon (Holmes et al., 2022; Marwick et al., 2015; Mayorga et al., 2005) due to a major input of aged biospheric OC from thawing permafrost (Wild et al., 2019; Hilton et al., 2015). The geodynamic setting of a river system also exerts a strong control on POC dynamics. On passive margins, terrigenous sediment typically experiences a series of erosion–deposition episodes because of the long distances between the upland source region and the ocean (Blair and Aller, 2012). Consequently, it is on active margins that the original POC source signature is transmitted with greater fidelity (Blair and Aller, 2012). Finally, humans greatly modify the delivery of fluvial sediment and associated POC to the ocean. In the last decade, sediment delivery in fluvial systems has increased by 215 %, whereas the net export of riverine sediment to the ocean has simultaneously decreased by 49 % (Syvitski et al., 2022), indicating the changing amount of eroded POC mobilized to fluvial systems and the final exported POC mass to the ocean (Stallard, 1998). While land-use change (e.g., soil erosion by agricultural practices) can lead to increasing terrestrial POC input (Syvitski et al., 2022; Dethier et al., 2022; Montgomery, 2007; Quinton et al., 2010), massive sequestration of POC upstream of dams significantly alters the nature and flux of downstream POC (Syvistski et al., 2022; Maavara et al., 2017; Best, 2019; Battin et al., 2009).
Even though recent research has advanced our understanding of the governing environmental factors from catchment to global scales (Galy et al., 2015; Hilton et al., 2008; Coppola et al., 2019; Hemingway et al., 2019; Eglinton et al., 2021), there is still a lack of quantitative constraints on the effect of environmental drivers on the carbon isotopic composition of riverine POC. The recent release of the International Soil Radiocarbon Database (ISRaD) (Lawrence et al., 2020) has enabled us to improve Earth system models aiming to predict global SOC radiocarbon distribution and turnover time (Shi et al., 2020; Carvalhais et al., 2014). However, such prediction is still hampered for fluvial POC, despite existing capabilities for modeling water discharge, SPM concentration, and POC content based on global water quality datasets (Ittekkot, 1988; Ludwig et al., 1996; Meybeck, 1993), such as the WBMsed global hydrology model (Cohen et al., 2014) or Global NEWS2 (Mayorga et al., 2010). Recently, owing to the improved water quality datasets, other sophisticated river biogeochemistry models have been built to understand riverine carbon cycling and environmental inturbations, such as the regional process-based Dynamic In-Stream Chemistry module (DISC-CARBON), but they still focus on different carbon fluxes (van Hoek et al., 2021).
Here we provide a new database for riverine POC, called MOREPOC (for MOdern River archivEs of Particulate Organic Carbon) v1.1, compiling 2283 Δ14C data, thereby representing a significant update of the previously reported global dataset by Marwick et al. (2015) with 531 reported Δ14C measurements. MOREPOC v1.1, featuring data published in international, peer-reviewed articles, provides the basis for (1) uncovering the fundamental mechanisms of preservation and alteration of river POC (in terms of “bulk” POC as well as for the individual POCbio and POCpetro pools) and (2) helping with the construction of numerical models able to simulate the isotopic compositions of POC in the context of global change. The MOREPOC database is publicly available on the Zenodo repository at https://doi.org/10.5281/zenodo.7055970 (Ke et al., 2022).
2.1 Data sources
In MOREPOC v1.1, through a comprehensive literature investigation of 115 peer-reviewed articles, we compiled 3546 POC-related data entries (each entry represents an individual sample), including 2195 with SPM concentration, 3053 with POC content, 3402 with stable carbon isotope δ13C values, 2283 with radiocarbon activity Δ14C values, 1937 with total nitrogen content (see details in Table 1), and 299 with aluminum-to-silicon mass ratios (Al Si). In addition, reported analytical uncertainties for POC content, δ13C, and Δ14C are included in MOREPOC. Note that riverbed or bank sediments are not included in this database. We selected studies reporting at least one carbon isotopic datum and those with paired elemental and dual carbon isotopic values. Studies reporting only POC contents were not compiled into MOREPOC v1.1. Potential mistakes generated during the compilation of data entries were carefully checked, and duplicate data were removed. A supplementary table, “MOREPOC_RM”, is provided to give additional information on the references, sampling method of SPM, filtration strategy, and carbonate removal methods and detailed information for the types of acid used to remove carbonate, etc.
2.2 Georeferencing
Locations of samples were digitalized if available, and an associate ArcGIS data layer in shapefile format (see MOREPOC_v1.1.rar) is provided with all points projected in a geographic coordinate system using the World Geodetic System 1984 (WGS1984). For references only providing a sampling map without any numerical information on the sampling location, sampling coordinates were manually extracted using ArcGIS 10.3 after georeferenced adjustment. In the end, 3339 SPM samples have coordinate information among the 3546 compiled SPM entries (Fig. 1). Furthermore, it can be noted that most studies chose sampling locations where SPM can be taken as representative of biogeochemical processes at catchment scale, i.e., the river mouth, to better understand the compositions, transport behavior, and fluxes of POC going either to a confluence or an estuary (e.g., Bouchez et al., 2014; Hilton et al., 2015; Holmes et al., 2022).
2.3 Database structure
To make MOREPOC v1.1 machine-readable, the compiled parameters were labeled with a short name as shown in Table 2.
2.4 Information on the sampling techniques
In the compiled studies, five different sampling techniques (parameter “type_spm” of MOREPOC v1.1) have been adopted to retrieve river sediments with the aim of measuring POC content and composition.
-
Surface SPM sampling (“type_spm = SS”) consists in collecting SPM within the first meter below the channel surface. This sampling scheme is the most frequently used and widely adopted in riverine POC studies.
-
Mid-depth SPM sampling (“MS”) consists in collecting SPM at an intermediate depth between the river surface and bottom. This sampling strategy has been used in studies on the Mekong (Martin et al., 2013) and Mackenzie (Campeau et al., 2020).
-
Integrated sampling over depth profiles (“ISD”) aims at obtaining a representative SPM sample accounting for grain size sorting along the water column, typically by making a flux-weighted composite of several samples collected at different depths along the water column. This sampling strategy has been adopted only for the Huanghe, Changjiang (Wang et al., 2012), and Zengjiang, a tributary of the Zhujiang (Gao et al., 2007).
-
Point sampling along depth profiles (“PSD”) is the collection of SPM along individual depth profiles at different depths in the water columns. In this method, and in contrast to the previous one, each SPM sample is treated and analyzed separately. This method allows access to the full range of particle sizes of SPM, explaining its wide use in the literature, e.g., Ganges–Brahmaputra (Galy et al., 2008a, b), Mackenzie (Hilton et al., 2015), and Bermejo (Repasch et al., 2021).
-
Point sampling over transects (“PST”) corresponds to PSD collection of SPM along several depth profiles across a given river channel section. This sophisticated sampling scheme allows for the exploration of the potential lateral heterogeneity in a river channel. It has recently been used in the Amazon (Bouchez et al., 2014), the Salween and the Irrawaddy (Baronas et al., 2020), and the Danube (Freymond et al., 2018).
2.5 Information on SPM extraction from river water samples and on analyzed size fractions
Broadly speaking, two methods are commonly adopted to extract SPM from a water sample: (1) continuous flow centrifugation, whereby large volumes of water can be centrifuged at high centrifugal forces; (2) filtration under pressure or vacuum using membranes made of glass fiber (GF/F), polyethersulfone (PES), polycarbonate (PTCE), nylon, quartz fiber, or mixed cellulose esters (MCEs), at a mesh size ranging from 0.2 to 1.0 µm. This information is recorded in MOREPOC_RM in detail if described in the corresponding source reference (parameter “Filters”).
In general, most studies used bulk SPM retrieved after filtration for the analysis of POC. However, in some studies, only certain size fractions of SPM were analyzed after separation into, e.g., a fine fraction (< 63 µm) and a coarse fraction (> 63 µm). This information is reported in MOREPOC v1.1 as the “fra_spm” parameter (see Table 2).
2.6 Information on the carbonate removal method
Particulate inorganic carbon (PIC) and POC have distinct carbon isotopic signatures, such that the accuracy of POC δ13C and Δ14C values could be compromised if the PIC is not efficiently removed by acidification prior to POC analysis (Komada et al., 2008). Three methods have been adopted in the studies referenced in MOREPOC v1.1:
-
the “acid rinse method”, in which sediment samples are soaked with diluted acid at a given temperature for a given time and then rinsed with distilled water;
-
the “acid vapor method”, in which sediment samples are exposed to vaporous concentrated hydrochloric acid in a closed system maintained at a given temperature for a given time and then evacuated under vacuum;
-
the “acid infiltration method”, in which sediment samples are infiltrated in situ in silver capsules with diluted hydrochloric acid and then subjected to drying.
The acid rinse method and acid vapor method have been widely used by the community to remove carbonates from sediments, and the “acid infiltration method” is also a common carbonate-removal method but is only reported by Menges et al. (2020) in MOREPOC v1.1.
In addition, a separate file of MOREPOC v1.1 (“MOREPOC_RM”) provides details on the carbonate removal method (m_acid), acid type (acid), molarity and quality (conc_acid), reaction time in hours (time_acid), and reaction temperature in degrees Celsius (temp_acid), allowing for quality evaluation of the method used in the cited references.
2.7 Definitions of POC composition variables and units
In MOREPOC v1.1, all data are either taken directly from the references or are calculated from the reference data. POC content (POC %) and total nitrogen content (TN %) are reported as dry weight percentage (%). In addition, POC concentration in river water (mg L−1) can be calculated using the SPM concentration reported as dry weight per liter (mg L−1) and percentage content of POC (%).
Most importantly, the fundamental component of MOREPOC v1.1 consists of an extensive dataset for stable carbon isotope values (δ13C, in ‰ relative to VPDB) and radiocarbon compositions (provided as both Δ14C in ‰ or as F14C; see below). Fraction modern, F14C, is the deviation of a sample's 14C atoms from that of the modern standard. Conventional radiocarbon ages (RCAs) are given in MOREPOC v1.1 following Stuiver and Polach (1977) using the Libby half-life of 5567 years with the mean life of 8033 for 14C. RCA is expressed in years before present (BP), with year zero being 1950:
The Δ14C value, which is defined as the relative difference between the absolute international standard (the base year 1950) and sample activity corrected for age and mass-dependent fractionation (Stuiver and Polach, 1977), is reported in MOREPOC v1.1 as well. A positive Δ14C indicates the presence of “bomb carbon”, whereas a negative Δ14C indicates that the radioactive decay of C overwhelms any incorporation of bomb carbon into the sample. The Δ14C calculation is defined as Eq. (2):
where yr is the year when the sediment was collected, and 8267 is the true mean life of 14C using the Cambridge half-life of 5730 years.
The term fraction of modern (F14C) is adopted in the above equations, and F14C is defined as Eq. (3) (Donahue et al., 1990):
where the denominator is 95 % of the 14C activity of the oxalic acid I (OxI) standard material in 1950, and the numerator is corrected for fractionation to a common δ13C value of −25 ‰.
Lastly, if available, the aluminum-to-silicon mass ratio (Al Si) is also provided in MOREPOC v1.1. This elemental ratio is an efficient proxy for the particle size of riverine sediment, allowing us to characterize the grain size effect of sediments on POC loading in fluvial delivery (Galy et al., 2008b; Bouchez et al., 2011; Hilton et al., 2015). The mineralogy and particle size of sediments are generally related, with coarse particles being quartz-rich (low Al Si ratios) and fine particles being clay-rich (high Al Si ratios) (Galy et al., 2008b). POC contents are usually positively correlated with proportions of fine-grained fractions (Mayer, 1994; Galy et al., 2008b; Bouchez et al., 2014).
2.8 Extent of MOREPOC v1.1
Although MOREPOC v1.1 features data from river systems worldwide, it does not offer the same degree of representativeness for all the continents, with, for instance, an overrepresentation of Asian rivers and an underrepresentation of rivers draining Europe and Oceania (Table 1). It can be noticed that there are relatively abundant POC studies on North American fluvial systems. However, the MOREPOC database also indicates the lack of studies on POC in fluvial systems in the cryosphere regions such as Antarctica and Greenland as well as in arid regions, including Australia and vast areas spanning from northern Africa to Middle Eastern Asia (Fig. 1).
The MOREPOC v1.1 database does not compile elemental and dual isotopic compositions of molecular compounds (plant-wax fatty acid and lignin phenol), thermal labile fractions, or black carbon. However, such complementary data could be incorporated into future versions.
3.1 Trends of δ13C and Δ14C in MOREPOC v1.1
The mobilization of terrestrial organic matter into fluvial systems depends on the interplay between tectonics, climate, geomorphology, lithology, and anthropogenic activities, all controlling to some extent the amount and composition of riverine POC (Blair et al., 2010; Eglinton et al., 2021).
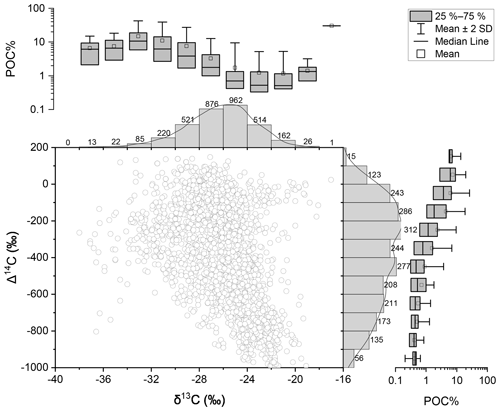
Figure 2δ13C versus Δ14C of MOREPOC v1.1 (n = 2,129). Frequency distribution histograms for δ13C (x axis) and Δ14C (y axis) are shown, with δ13C values binned every −2.5 ‰ from −40 ‰ to −15 ‰ and Δ14C values binned every −50 ‰ from −1000 ‰ to 200 ‰. Each bin is labeled with the number of samples it hosts. Solid lines represent the corresponding probability density functions. Box charts represent the statistical analysis of POC % in each bin of δ13C (x axis) and Δ14C (y axis).
Riverine POC displays significant heterogeneity in elemental and isotopic compositions of carbon around the globe (Figs. 1 and 2). δ13C values (n = 3402) range from −38 ‰ to −17 ‰, with an average value of −26.3 ‰. As shown in Fig. 2, the majority of the data fall between −28 ‰ and −24 ‰ (n = 1770, 52.0 % of total entries), which is consistent with the overall isotopic signature of the terrestrial biosphere of −26 ± 7 ‰ (Schidlowski, 1988). The ages of riverine POC span from “modern” (that is, recording bomb-derived carbon) to “ancient” (strongly influenced by fossil petrogenic sources), with Δ14C values (n = 2283) ranging from −990.1 ‰ to 147.7 ‰ with a statistical average of −386 ‰. A large fraction of the Δ14C values (n = 775, 33.9 % of total entries) falls within the range −300 ‰ to 0 ‰; this range dominates the database in Marwick et al. (2015) as well (n = 278, 52.3 % of Marwick's total entries). The MOREPOC v1.1 dataset is on average more 14C-depleted than that of Marwick et al. (2015).
Around the globe, the most ancient POC (Δ14C = −990 ‰) is found in small mountainous rivers in Taiwan (Hilton et al., 2010), in which the entirety of POC is derived from the erosion of sedimentary rocks. In the riverine POC dataset of MOREPOC v1.1, bomb carbon signals are abundant (Δ14C > 0 ‰), particularly for African rivers in tropical regions such as the Athi-Galana-Sabaki, Tana, Zambezi, and Congo (Marwick et al., 2015; Spencer et al., 2012), rivers in North America, including the Hudson, Siuslaw, and York, rivers draining to the Hudson Bay (Leithold et al., 2006; Raymond and Bauer, 2001; Godin et al., 2017; Longworth et al., 2007), and the Andean Amazon (Mayorga et al., 2005; Townsend-Small et al., 2007). Around the Qinghai–Tibet Plateau, where most large river systems in eastern and southern Asia share similar high-elevation source regions, POC is usually characterized by relatively depleted 14C signals due to high erosion rates of sedimentary rocks in mountainous regions, like in the Ganges–Brahmaputra (Galy et al., 2007) or Changjiang (Wang et al., 2012, 2019), and erosion of soils containing pre-aged OC, e.g., Huanghe (Tao et al., 2015). The most depleted 13C signatures (less than −35 ‰) are observed for POC from Arctic rivers, such as the Ob', Yukon, and Kolyma (Holmes et al., 2022). The highest δ13C values (higher than −22 ‰) are found in rivers in Africa (Athi-Galana-Sabaki, Betsiboka, and Tana; Marwick et al., 2015; Tamooh et al., 2013) and mountainous rivers, e.g., Taiwan (Hilton et al., 2010), the upper Ganges (Galy et al., 2007, 2008b), and Minjiang (Wang et al., 2019).
As observed in the global compilation in Fig. 2, elemental and isotopic data of POC generally show an inverse relationship between δ13C and Δ14C and generally an increasing POC content with higher radiocarbon activity of POC. Indeed, OC from sedimentary rocks (i.e., dead OC with Δ14C = −1000 ‰ by definition) usually has 13C-enriched signatures compared to recent biomass. Eroded material from sedimentary rocks thus has lower POC content, 14C-depleted signatures, and relatively high δ13C signatures. This global pattern stems from the global dominance of C3 plants in the compiled catchments (Fig. 2). However, POC-rich riverine SPM can also be relatively enriched in 13C, i.e., δ13C values larger than −20 ‰ (Figs. 2 and 3). This pattern indicates the presence of an additional pool of 14C- and 13C-rich POC in the terrestrial environment (Cerling et al., 1997), consisting of modern C4 plants in catchments dominated by grasslands or savannah (e.g., Marwick et al., 2015). The maximum values of δ13C and Δ14C of POC (dotted line in Fig. 3) tend to be more depleted at high latitudes than at low latitudes. This might reflect the major POC components. (1) Dominated by POCbio, the combined effects of increasing coverage of C4 plants in tropical regions and the input of pre-aged OCbio of C3 plants from degrading permafrost at high latitude (Cerling et al., 1997; Still et al., 2003). (2) Dominated by POCpetro, rivers in mountainous regions tend to erode 13C-enriched petrogenic OC (Hilton et al., 2010; Galy et al., 2007). In addition, aquatic authigenic production can be an important mechanism contributing 13C-depleted and 14C-enriched POC (Longworth et al., 2007; Marwick et al., 2015; Wu et al., 2018).
3.2 Relationships between riverine SPM and POC
MOREPOC v1.1 features data from rivers with SPM concentrations ranging from 0.35 to 199 000 mg L−1, with POC content from 0.01 % to 91.67 %. SPM and POC concentrations (both mg L−1; n = 2115) are positively correlated (Fig. 4). However, the global trend shows that an increase in SPM concentration is accompanied by a decrease in POC content (%), which is largely due to a dilution effect by inorganic materials (Fig. 4, Ittekkot, 1988; Ludwig et al., 1996; Meybeck, 1993). In MOREPOC v1.1, large SPM concentrations (over 10 000 mg L−1) are generally observed in mountainous rivers, such as the Choshui and Liwu rivers in Taiwan (Hilton et al., 2008; Kao et al., 2014), the Santa Clara River (USA) (Masiello and Druffel, 2001), or the Minjiang (a major tributary of the upper Changjiang, China) (Wang et al., 2019). The Huanghe River is an exception in that it has very large SPM concentrations in its middle reaches where it drains the Chinese Loess Plateau (Qu et al., 2020; Hu et al., 2015). Although the sediment of highly turbid rivers is typically POC-poor, high sediment concentrations generate the largest POC export rates (Fig. 4). This observation also underlines the importance of sediment transport near the channel bottom in large rivers where SPM concentration is usually much higher than at the surface (Fig. 5), e.g., Ganges–Brahmaputra–Meghna (Galy et al., 2007, 2008b), Mackenzie (Hilton et al., 2015), Amazon (Bouchez et al., 2014), and Yukon (Holmes et al., 2022), as well as the role of stochastic events leading to high-turbidity episodes such as storms, landslides, or earthquakes (Hilton et al., 2008; Wang et al., 2015; Frith et al., 2018). Small SPM concentrations (less than 10 mg L−1) are generally found in rivers during the frozen season or rivers draining either high-latitude or tropical areas characterized by low-relief settings, in which POC content is relatively high (Gao et al., 2007; Holmes et al., 2022).
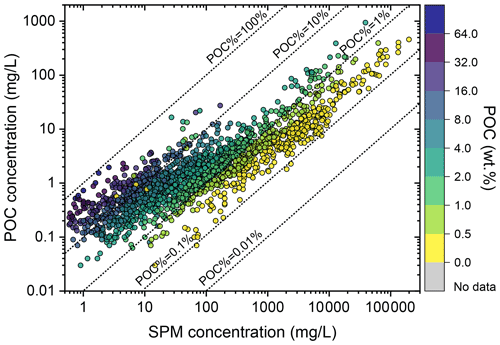
Figure 4River SPM concentration versus POC concentration (n = 2135) (both mg L−1). Dotted lines represent contours of constant POC content. Colors indicate the POC content from data entries in MOREPOC v1.1.
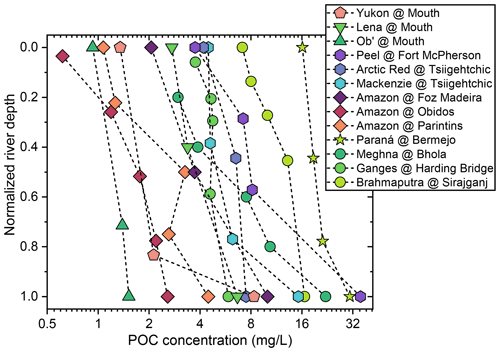
Figure 5POC concentration variation in vertical water columns from depth profiles in global large rivers. Selected depth profiles are from the Yukon, Lena, and Ob' (Holmes et al., 2022), Mackenzie including Peel and Arctic Red (Hilton et al., 2015), Amazon (Bouchez et al., 2014), Paraná (Repasch et al., 2021), and Ganges–Brahmaputra–Meghna systems (Galy et al., 2007, 2008b). Normalized river depth is calculated by normalizing the individual sample depth to the maximum sample depth of the corresponding profile.
In general, POC becomes 14C-depleted with increasing suspended sediment load and decreasing POC content. These patterns are most likely caused by the dilution of POCbio by POCpetro in areas of strong erosion (Leithold et al., 2016). However, MOREPOC v1.1 also highlights that the low SPM load associated with high POC content is often characterized by significantly low Δ14C values (Figs. 6, 7). Most of those samples come from Arctic river systems. This raises some concerns because Arctic permafrost soils store approximately twice the current amount of carbon contained in the atmosphere (Zimov et al., 2006), and biospheric OC that was previously stored in frozen soils over thousands of years is being released and can induce accelerated environmental changes (Schuur et al., 2015; Vonk et al., 2015; Wild et al., 2019). How OC in permafrost regions responds to global warming should be a key research issue in future studies. Meanwhile, it is worth noting, for a given SPM concentration, that 14C abundance can be contrasted. For example, rivers draining low-latitude, tropical regions (especially 10∘ N–10∘ S; e.g., African rivers) or high-latitude regions (60–75∘ N; e.g., Siberian Arctic rivers) are usually characterized by relatively low SPM concentration and abundant POC composition. Nevertheless, riverine POC from the low-latitude African rivers is much younger compared to that from the Arctic Siberian regions. This difference most likely stems from the contrasting radiocarbon activities and turnover times of the soil organic carbon between these two regions, which are primarily driven by climate (Eglinton et al., 2021; Marwick et al., 2015; Wild et al., 2019; Vonk et al., 2015).
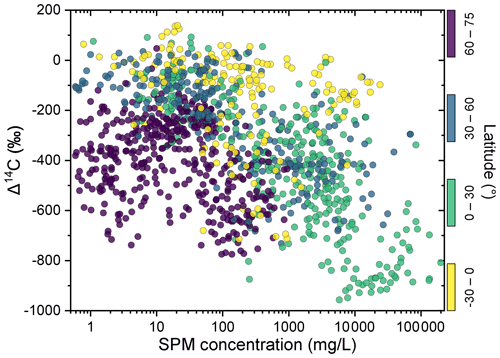
Figure 6POC Δ14C versus SPM concentration (n = 1157). Colors indicate the latitude of the sampling location. Note the log scale used for the SPM concentration.
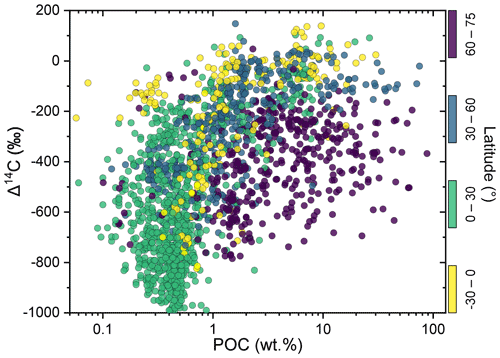
Figure 7Δ14C values versus POC content (n = 1860). Colors indicate the latitude of the sampling location. Note the log scale used for the POC content.
The MOREPOC v1.1 dataset also reveals that, under a given climate, river systems can be heterogeneous in terms of SPM concentration and the associated POC composition. For example, amongst circum-Arctic rivers, the Mackenzie River has a relatively large SPM concentration of 135.1 mg L−1 on average (1σ = 16.6, n = 106), with 3.01 % POC (1σ = 0.39, n = 105) characterized by a fairly low Δ14C (average value of −599.5 ‰, 1σ = 7.7, n = 118) near the river mouth (Hilton et al., 2015; Schwab et al., 2020; Holmes et al., 2022). In contrast, the Yenisei River only has an average SPM concentration of 5.2 mg L−1 (1σ = 0.6, n = 86) but much higher POC contents (18.0 %, 1σ = 2.1, n = 83) and Δ14C values (−342.3 ‰, 1σ = 15.7, n = 66; Holmes et al., 2022). Such a difference suggests that lithology and geomorphology can play an important role in riverine POC composition and load by providing a substantial fraction of fossil OC (Hilton et al., 2015). On the other hand, small mountainous rivers such as those in Taiwan or those draining the Himalayas show large SPM concentrations and low POC contents with low radiocarbon activities. These regions characterized by active tectonics, steep slopes, and intense precipitation act as global hotspots for sediment production and thus petrogenic OC mobilization (Milliman and Farnsworth, 2011; Hilton and West, 2020).
The MOREPOC v1.1 database is publicly available on the Zenodo repository in machine-readable formats as an Excel spreadsheet (.xslx), a comma-limited table (.csv), and a GIS shapefile at https://doi.org/10.5281/zenodo.7055970 (Ke et al., 2022).
In this paper, we introduce MOREPOC, the largest and most comprehensive database for riverine suspended particulate matter (SPM) concentration and particulate organic carbon (POC) composition, including POC and total nitrogen (TN) content, stable carbon isotope (13C), cosmogenic–radioactive carbon isotope (14C), as well as aluminum-to-silicon (Al Si) mass ratios. MOREPOC will benefit the scientific community carrying out research on riverine POC sources, transport, and fate. Furthermore, it will help feed and validate Earth system models in order to improve the ability of models to constrain all the components of the global carbon cycle. Combined with ocean sediment databases, such as CASCADE (Circum-Arctic Sediment Carbon DatabasE; Martens et al., 2021) or MOSAIC (Modern Ocean Sediment Archive and Inventory of Carbon; Van der Voort et al., 2021), MOREPOC will enable a better understanding of the fate of POC from the terrestrial source to sink at the ocean bottom. Existing environmental raster global datasets for climate, geomorphology, lithology, tectonics, hydrology, and land use also offer promising prospects for the use of MOREPOC for identifying the controls on POC fluxes and composition, in particular using advanced statistical analysis or machine-learning techniques. Future updates of MOREPOC should include new bulk POC parameters as well as data on molecular fractions, thermal labile fractions, or specific components such as black carbon or fossil carbon, which should, in turn, provide additional insight into the alteration of riverine POC from source to sink, an essential feature of the global carbon cycle.
YK collected the MOREPOC data and conceptualized, designed, structured, and filled the database. YK and DC contributed to the database checking. YK prepared the manuscript. YK drafted and coordinated the manuscript with input from DC, JB, and CQ.
The contact author has declared that none of the authors has any competing interests.
Publisher’s note: Copernicus Publications remains neutral with regard to jurisdictional claims in published maps and institutional affiliations.
The development of the MOREPOC database was funded by the Agence Nationale de la Recherche (ANR) SEDIMAN (grant no. ANR-15-CE01-0012). Yutian Ke was supported by the China Scholarship Council with a PhD scholarship (grant no. 201706180008).
This research has been supported by the Agence Nationale de la Recherche (grant no. ANR-15-CE01-0012) and the China Scholarship Council (grant no. 201706180008).
This paper was edited by Attila Demény and reviewed by Jin Wang and three anonymous referees.
Baronas, J. J., Stevenson, E. I., Hackney, C. R., Darby, S. E., Bickle, M. J., Hilton, R. G., Larkin, C. S., Parsons, D. R., Khaing, A. M., and Tipper, E. T.: Integrating suspended sediment flux in large alluvial river channels: application of a synoptic Rouse-based model to the Irrawaddy and Salween rivers, J. Geophys. Res., 125, e2020JF00555, https://doi.org/10.1029/2020JF005554, 2020.
Battin, T. J., Luyssaert, S., Kaplan, L. A., Aufdenkampe, A. K., Richter, A., and Tranvik, L. J.: The boundless carbon cycle, Nat. Geosci., 2, 598–600, https://doi.org/10.1038/ngeo618, 2009.
Berner, R. A.: The long-term carbon cycle, fossil fuels and atmospheric composition, Nature, 426, 323–326, https://doi.org/10.1038/nature02131, 2003.
Best, J.: Anthropogenic stresses on the world's big rivers, Nat. Geosci., 12, 7–21, https://doi.org/10.1038/s41561-018-0262-x, 2019.
Blair, N. E. and Aller, R. C.: The fate of terrestrial organic carbon in the marine environment., Ann. Rev. Mar. Sci., 4, 401–423, https://doi.org/10.1146/annurev-marine-120709-142717, 2012.
Blair, N. E., Leithold, E. L., Ford, S. T., Peeler, K. A., Holmes, J. C., and Perkey, D. W.: The persistence of memory: The fate of ancient sedimentary organic carbon in a modern sedimentary system, Geochim. Cosmochim. Acta, 67, 63–73, https://doi.org/10.1016/S0016-7037(02)01043-8, 2003.
Blair, N. E., Leithold, E. L., and Aller, R. C.: From bedrock to burial: the evolution of particulate organic carbon across coupled watershed-continental margin systems, Mar. Chem., 92, 141–156, https://doi.org/10.1016/j.marchem.2004.06.023, 2004.
Blair, N. E., Leithold, E. L., Brackley, H., Trustrum, N., Page, M., and Childress, L.: Terrestrial sources and export of particulate organic carbon in the Waipaoa sedimentary system: Problems, progress and processes, Mar. Geol., 270, 108–118, https://doi.org/10.1016/j.margeo.2009.10.016, 2010.
Bouchez, J., Gaillardet, J., France-Lanord, C., Maurice, L., and Dutra-maia, P.: Grain size control of river suspended sediment geochemistry: Clues from Amazon River depth profiles, Geochem. Geophy. Geosy., 12, Q03008, https://doi.org/10.1029/2010GC003380, 2011.
Bouchez, J., Galy, V., Hilton, R. G., Gaillardet, J., Moreira-Turcq, P., Pérez, M. A., France-Lanord, C., and Maurice, L.: Source, transport and fluxes of Amazon River particulate organic carbon: Insights from river sediment depth-profiles, Geochim. Cosmochim. Acta, 133, 280–298, 2014.
Burdige, D. J.: Burial of terrestrial organic matter in marine sediments: A re-assessment, Global Biogeochem. Cy., 19, GB4011, https://doi.org/10.1029/2004gb002368, 2005.
Campeau, A., Soerensen, A. L., Martma, T., Åkerblom, S., and Zdanowicz, C.: Controls on the 14C content of dissolved and particulate organic carbon mobilized across the Mackenzie River basin, Canada, Global Biogeochem. Cy., 34, e2020GB006671, https://doi.org/10.1029/2020GB006671, 2020.
Carvalhais, N., Forkel, M., Khomik, M., Bellarby, J., Jung, M., Migliavacca, M., Saatchi, S., Santoro, M., Thurner, M., and Weber, U.: Global covariation of carbon turnover times with climate in terrestrial ecosystems, Nature, 514, 213–217, https://doi.org/10.1038/nature13731, 2014.
Cerling, T. E., Harris, J. M., MacFadden, B. J., Leakey, M. G., Quade, J., Eisenmann, V., and Ehleringer, J. R.: Global vegetation change through the Miocene/Pliocene boundary, Nature, 389, 153–158, https://doi.org/10.1038/38229, 1997.
Cohen, S., Kettner, A. J., and Syvitski, J. P. M.: Global suspended sediment and water discharge dynamics between 1960 and 2010: Continental trends and intra-basin sensitivity, Glob. Planet. Change, 115, 44–58, https://doi.org/10.1016/j.gloplacha.2014.01.011, 2014.
Coppola, A. I., Wiedemeier, D. B., Galy, V., Haghipour, N., Hanke, U. M., Nascimento, G. S., Usman, M., Blattmann, T. M., Reisser, M., Freymond, C. V., Zhao, M. X., Voss, B., Wacker, L., Schefuß, E., Peucker-Ehrenrink, B., Abiven, Samuel., Schmidt, M. W., and Eglinton, T. I.: Global-scale evidence for the refractory nature of riverine black carbon, Nat. Geosci., 11, 584–588, https://doi.org/10.1038/s41561-018-0159-8, 2019.
Dethier, E. N., Renshaw, C. E., and Magilligan, F. J.: Rapid changes to global river suspended sediment flux by humans, Science, 376, 1447–1452, https://doi.org/10.1126/science.abn7980, 2022.
Donahue, D. J., Linick, T. W., and Jull, A. T.: Isotope-Ratio and Background Corrections for Accelerator Mass Spectrometry Radiocarbon Measurements, Radiocarbon, 32, 135–142, https://doi.org/10.1017/S0033822200040121, 1990.
Eglinton, T. I., Galy, V. V, Hemingway, J. D., Feng, X., Bao, H., Blattmann, T. M., Dickens, A. F., Gies, H., Giosan, L., and Haghipour, N.: Climate control on terrestrial biospheric carbon turnover, P. Natl. Acad. Sci. USA, 118, e2011585118, https://doi.org/10.1073/pnas.2011585118, 2021.
Freymond, C. V., Lupker, M., Peterse, F., Haghipour, N., Wacker, L., Filip, F., Giosan, L., and Eglinton, T. I.: Constraining Instantaneous Fluxes and Integrated Compositions of Fluvially Discharged Organic Matter, Geochem. Geophy. Geosy., 19, 2453–2462, https://doi.org/10.1029/2018GC007539, 2018.
Frith, N. V., Hilton, R. G., Howarth, J. D., Gröcke, D. R., Fitzsimons, S. J., Croissant, T., Wang, J., McClymont, E. L., Dahl, J., and Densmore, A. L.: Carbon export from mountain forests enhanced by earthquake-triggered landslides over millennia, Nat. Geosci., 11, 772–776, https://doi.org/10.1038/s41561-018-0216-3, 2018.
Galy, V. and Eglinton, T.: Protracted storage of biospheric carbon in the Ganges-Brahmaputra basin, Nat. Geosci., 4, 843–847, https://doi.org/10.1038/ngeo1293, 2011.
Galy, V., France-Lanord, C., Beyssac, O., Faure, P., Kudrass, H., and Palhol, F.: Efficient organic carbon burial in the Bengal fan sustained by the Himalayan erosional system, Nature, 450, 407–410, https://doi.org/10.1038/nature06273, 2007.
Galy, V., Beyssac, O., France-Lanord, C., and Eglinton, T.: Recycling of Graphite During Himalayan Erosion: A Geological Stabilization of Carbon in the Crust, Science, 322, 943–945, https://doi.org/10.1126/science.1161408, 2008a.
Galy, V., France-Lanord, C., and Lartiges, B.: Loading and fate of particulate organic carbon from the Himalaya to the Ganga–Brahmaputra delta, Geochim. Cosmochim. Acta, 72, 1767–1787, https://doi.org/10.1016/j.gca.2008.01.027, 2008b.
Galy, V., Peucker-Ehrenbrink, B., and Eglinton, T. I.: Global carbon export from the terrestrial biosphere controlled by erosion, Nature, 521, 204–207, https://doi.org/10.1038/nature14400, 2015.
Gao, Q., Tao, Z., Yao, G., Ding, J., Liu, Z., and Liu, K.: Elemental and isotopic signatures of particulate organic carbon in the Zengjiang River, southern China, Hydrol. Process. An Int. J., 21, 1318–1327, https://doi.org/10.1002/hyp.6358, 2007.
Godin, P., Macdonald, R. W., Kuzyk, Z. Z. A., Goñi, M. A., and Stern, G. A.: Organic matter compositions of rivers draining into Hudson Bay: Present-day trends and potential as recorders of future climate change, J. Geophys. Res.-Biogeo., 122, 1848–1869, https://doi.org/10.1002/2016JG003569, 2017.
Hemingway, J. D., Rothman, D. H., Grant, K. E., Rosengard, S. Z., Eglinton, T. I., Derry, L. A., and Galy, V. V: Mineral protection regulates long-term global preservation of natural organic carbon, Nature, 570, 228–231, https://doi.org/10.1038/s41586-019-1280-6, 2019.
Hilton, R. G. and West, A. J.: Mountains, erosion and the carbon cycle, Nat. Rev. Earth Environ., 1, 284–299, https://doi.org/10.1038/s43017-020-0058-6, 2020.
Hilton, R. G., Galy, A., Hovius, N., Chen, M.-C., Horng, M.-J., and Chen, H.: Tropical-cyclone-driven erosion of the terrestrial biosphere from mountains, Nat. Geosci., 1, 759–762, https://doi.org/10.1038/ngeo333, 2008.
Hilton, R. G., Galy, A., Hovius, N., Horng, M.-J., and Chen, H.: The isotopic composition of particulate organic carbon in mountain rivers of Taiwan, Geochim. Cosmochim. Ac., 74, 3164–3181, https://doi.org/10.1016/j.gca.2010.03.004, 2010.
Hilton, R. G., Galy, A., Hovius, N., Horng, M.-J., and Chen, H.: Efficient transport of fossil organic carbon to the ocean by steep mountain rivers: An orogenic carbon sequestration mechanism, Geology, 39, 71–74, https://doi.org/10.1130/G31352.1, 2011.
Hilton, R. G., Gaillardet, J., Calmels, D., and Birck, J.-L.: Geological respiration of a mountain belt revealed by the trace element rhenium, Earth Planet. Sci. Lett., 403, 27–36, https://doi.org/10.1016/j.epsl.2014.06.021, 2014.
Hilton, R. G., Galy, V., Gaillardet, J., Dellinger, M., Bryant, C., O'Regan, M., Gröcke, D. R., Coxall, H., Bouchez, J., and Calmels, D.: Erosion of organic carbon in the Arctic as a geological carbon dioxide sink, Nature, 524, 84–87, https://doi.org/10.1038/nature14653, 2015.
Holmes, R. M., McClelland, J. W., Tank, S. E., Spencer, R. G. M., and Shiklomanov, A. I.: Water Quality Dataset, Arctic Great Rivers Observatory, Version 20220609, https://www.arcticgreatrivers.org/data, last access: 9 June 2022.
Hu, B., Li, J., Bi, N., Wang, H., Wei, H., Zhao, J., Xie, L., Zou, L., Cui, R., Li, S., Liu, M., and Li, G.: Effect of human-controlled hydrological regime on the source, transport, and flux of particulate organic carbon from the lower Huanghe (Yellow River), Earth Surf. Proc. Land., 40, 1029–1042, https://doi.org/10.1002/esp.3702, 2015.
Ittekkot, V.: Global trends in the nature of organic matter in river suspensions, Nature, 332, 436–438, 1988.
Kao, S.-J., Hilton, R. G., Selvaraj, K., Dai, M., Zehetner, F., Huang, J.-C., Hsu, S.-C., Sparkes, R., Liu, J. T., Lee, T.-Y., Yang, J.-Y. T., Galy, A., Xu, X., and Hovius, N.: Preservation of terrestrial organic carbon in marine sediments offshore Taiwan: mountain building and atmospheric carbon dioxide sequestration, Earth Surf. Dynam., 2, 127–139, https://doi.org/10.5194/esurf-2-127-2014, 2014.
Ke, Y. T., Calmels, D., Bouchez, J., and Quantin, C.: MOdern River archivEs of Particulate Organic Carbon: MOREPOC (1.1), Zenodo [data set], https://doi.org/10.5281/zenodo.7055970, 2022.
Komada, T., Anderson, M. R., and Dorfmeier, C. L.: Carbonate removal from coastal sediments for the determination of organic carbon and its isotopic signatures, δ13C and Δ14C: comparison of fumigation and direct acidification by hydrochloric acid, Limnol. Oceanogr. Methods, 6, 254–262, https://doi.org/10.4319/lom.2008.6.254, 2008.
Lawrence, C. R., Beem-Miller, J., Hoyt, A. M., Monroe, G., Sierra, C. A., Stoner, S., Heckman, K., Blankinship, J. C., Crow, S. E., McNicol, G., Trumbore, S., Levine, P. A., Vindušková, O., Todd-Brown, K., Rasmussen, C., Hicks Pries, C. E., Schädel, C., McFarlane, K., Doetterl, S., Hatté, C., He, Y., Treat, C., Harden, J. W., Torn, M. S., Estop-Aragonés, C., Asefaw Berhe, A., Keiluweit, M., Della Rosa Kuhnen, Á., Marin-Spiotta, E., Plante, A. F., Thompson, A., Shi, Z., Schimel, J. P., Vaughn, L. J. S., von Fromm, S. F., and Wagai, R.: An open-source database for the synthesis of soil radiocarbon data: International Soil Radiocarbon Database (ISRaD) version 1.0, Earth Syst. Sci. Data, 12, 61–76, https://doi.org/10.5194/essd-12-61-2020, 2020.
Leithold, E. L., Blair, N. E., and Perkey, D. W.: Geomorphologic controls on the age of particulate organic carbon from small mountainous and upland rivers, Global Biogeochem. Cy., 20, GB3022, https://doi.org/10.1029/2005GB002677, 2006.
Leithold, E. L., Blair, N. E., and Wegmann, K. W.: Source-to-sink sedimentary systems and global carbon burial: A river runs through it, Earth-Sci. Rev., 153, 30–42, https://doi.org/10.1016/j.earscirev.2015.10.011, 2016.
Longworth, B. E., Petsch, S. T., Raymond, P. A., and Bauer, J. E.: Linking lithology and land use to sources of dissolved and particulate organic matter in headwaters of a temperate, passive-margin river system, Geochim. Cosmochim. Acta, 71, 4233–4250, https://doi.org/10.1016/j.gca.2007.06.056, 2007.
Ludwig, W., Probst, J.-L., and Kempe, S.: Predicting the oceanic input of organic carbon by continental erosion, Global Biogeochem. Cy., 10, 23–41, https://doi.org/10.1029/95GB02925, 1996.
Maavara, T., Lauerwald, R., Regnier, P., and Van Cappellen, P.: Global perturbation of organic carbon cycling by river damming, Nat. Commun., 8, 15347, https://doi.org/10.1038/ncomms15347, 2017.
Martens, J., Romankevich, E., Semiletov, I., Wild, B., van Dongen, B., Vonk, J., Tesi, T., Shakhova, N., Dudarev, O. V., Kosmach, D., Vetrov, A., Lobkovsky, L., Belyaev, N., Macdonald, R. W., Pieńkowski, A. J., Eglinton, T. I., Haghipour, N., Dahle, S., Carroll, M. L., Åström, E. K. L., Grebmeier, J. M., Cooper, L. W., Possnert, G., and Gustafsson, Ö.: CASCADE – The Circum-Arctic Sediment CArbon DatabasE, Earth Syst. Sci. Data, 13, 2561–2572, https://doi.org/10.5194/essd-13-2561-2021, 2021.
Martin, E. E., Ingalls, A. E., Richey, J. E., Keil, R. G., Santos, G. M., Truxal, L. T., Alin, S. R., and Druffel, E. R. M.: Age of riverine carbon suggests rapid export of terrestrial primary production in tropics, Geophys. Res. Lett., 40, 5687–5691, https://doi.org/10.1002/2013GL057450, 2013.
Marwick, T. R., Tamooh, F., Teodoru, C. R., Borges, A. V, Darchambeau, F., and Bouillon, S.: The age of river transported carbon: A global perspective, Global Biogeochem. Cy., 29, 122–137, https://doi.org/10.1002/2014GB004911, 2015.
Masiello, C. A. and Druffel, E. R. M.: Carbon isotope geochemistry of the Santa Clara River, Global Biogeochem. Cy., 15, 407–416, https://doi.org/10.1029/2000GB001290, 2001.
Mayer, L. M.: Surface area control of organic carbon accumulation in continental shelf sediments, Geochim. Cosmochim. Acta, 58, 1271–1284, https://doi.org/10.1016/0016-7037(94)90381-6, 1994.
Mayorga, E., Aufdenkampe, A. K., Masiello, C. A., Krusche, A. V, Hedges, J. I., Quay, P. D., Richey, J. E., and Brown, T. A.: Young organic matter as a source of carbon dioxide outgassing from Amazonian rivers, Nature, 436, 538–541, https://doi.org/10.1038/nature03880, 2005.
Mayorga, E., Seitzinger, S. P., Harrison, J. A., Dumont, E., Beusen, A. H. W., Bouwman, A. F., Fekete, B. M., Kroeze, C., and Van Drecht, G.: Global nutrient export from WaterSheds 2 (NEWS 2): model development and implementation, Environ. Model. Softw., 25, 837–853, https://doi.org/10.1016/j.envsoft.2010.01.007, 2010.
Meybeck, M.: Riverine transport of atmospheric carbon: sources, global typology and budget, Water. Air. Soil Pollut., 70, 443–463, https://doi.org/10.1007/BF01105015, 1993.
Milliman, J. D. and Farnsworth, K. L.: River discharge to the coastal ocean: a global synthesis, Cambridge University Press, ISBN 978-0-521-87987-3, 2011.
Montgomery, D. R.: Soil erosion and agricultural sustainability, P. Natl. Acad. Sci. USA, 104, 13268–13272, https://doi.org/10.1073/pnas.0611508104, 2007.
Petsch, S. T.: Weathering of organic carbon, Treatise on Geochemistry, 2nd edn., Elsevier Science, 12, 217–238, https://doi.org/10.1016/B978-0-08-095975-7.01013-5, 2014.
Qu, Y., Jin, Z., Wang, J., Wang, Y., Xiao, J., Gou, L., Zhang, F., Liu, C., Gao, Y., Suarez, M. B., and Xu, X.: The sources and seasonal fluxes of particulate organic carbon in the Yellow River, Earth Surf. Proc. Land., 45, 2004–2019, https://doi.org/10.1002/esp.4861, 2020.
Quinton, J. N., Govers, G., Van Oost, K., and Bardgett, R. D.: The impact of agricultural soil erosion on biogeochemical cycling, Nat. Geosci., 3, 311–314, https://doi.org/10.1038/ngeo838, 2010.
Raymond, P. A. and Bauer, J. E.: Riverine export of aged terrestrial organic matter to the North Atlantic Ocean, Nature, 409, 497–500, https://doi.org/10.1038/35054034, 2001.
Repasch, M., Scheingross, J. S., Hovius, N., Lupker, M., Wittmann, H., Haghipour, N., Gröcke, D. R., Orfeo, O., Eglinton, T. I., and Sachse, D.: Fluvial organic carbon cycling regulated by sediment transit time and mineral protection, Nat. Geosci., 14, 842–848, https://doi.org/10.1038/s41561-021-00845-7, 2021.
Schwab, M. S., Hilton, R. G., Raymond, P. A., Haghipour, N., Amos, E., Tank, S. E., Holmes, R. M., Tipper, E. T., and Eglinton, T. I.: An Abrupt Aging of Dissolved Organic Carbon in Large Arctic Rivers, Geophys. Res. Lett., 47, e2020GL088823, https://doi.org/10.1029/2020gl088823, 2020.
Schidlowski, M.: A 3,800-million-year isotopic record of life from carbon in sedimentary rocks, Nature, 333, 313–318, https://doi.org/10.1038/333313a0, 1988.
Schuur, E. A., McGuire, A. D., Schadel, C., Grosse, G., Harden, J. W., Hayes, D. J., Hugelius, G., Koven, C. D., Kuhry, P., Lawrence, D. M., Natali, S. M., Olefeldt, D., Romanovsky, V. E., Schaefer, K., Turetsky, M. R., Treat, C. C., and Vonk, J. E.: Climate change and the permafrost carbon feedback, Nature, 520, 171–179, https://doi.org/10.1038/nature14338, 2015.
Shi, Z., Allison, S. D., He, Y., Levine, P. A., Hoyt, A. M., Beem-Miller, J., Zhu, Q., Wieder, W. R., Trumbore, S., and Randerson, J. T.: The age distribution of global soil carbon inferred from radiocarbon measurements, Nat. Geosci., 13, 555–559, https://doi.org/10.1038/s41561-020-0596-z, 2020.
Spencer, R. G. M., Hernes, P. J., Aufdenkampe, A. K., Baker, A., Gulliver, P., Stubbins, A., Aiken, G. R., Dyda, R. Y., Butler, K. D., and Mwamba, V. L.: An initial investigation into the organic matter biogeochemistry of the Congo River, Geochim. Cosmochim. Acta, 84, 614–627, https://doi.org/10.1016/j.gca.2012.01.013, 2012.
Stallard, R. F.: Terrestrial sedimentation and the carbon cycle: Coupling weathering and erosion to carbon burial, Global Biogeochem. Cy., 12, 231–257, https://doi.org/10.1029/98GB00741, 1998.
Still, C. J., Berry, J. A., Collatz, G. J., and DeFries, R. S.: Global distribution of C3 and C4 vegetation: carbon cycle implications, Global Biogeochem. Cy., 17, 1–6, https://doi.org/10.1029/2001GB001807, 2003.
Stuiver, M. and Polach, H. A.: Discussion reporting of 14C data, Radiocarbon, 19, 355–363, https://doi.org/10.1017/S0033822200003672, 1977.
Syvitski, J., Ángel, J. R., Saito, Y., Overeem, I., Vörösmarty, C. J., Wang, H., and Olago, D.: Earth's sediment cycle during the Anthropocene, Nat. Rev. Earth Environ., 3, 179–196, https://doi.org/10.1038/s43017-021-00253-w, 2022.
Tamooh, F., Borges, A. V., Meysman, F. J. R., Van Den Meersche, K., Dehairs, F., Merckx, R., and Bouillon, S.: Dynamics of dissolved inorganic carbon and aquatic metabolism in the Tana River basin, Kenya, Biogeosciences, 10, 6911–6928, https://doi.org/10.5194/bg-10-6911-2013, 2013.
Tao, S., Eglinton, T. I., Montluçon, D. B., McIntyre, C., and Zhao, M.: Pre-aged soil organic carbon as a major component of the Yellow River suspended load: Regional significance and global relevance, Earth Planet. Sci. Lett., 414, 77–86, https://doi.org/10.1016/j.epsl.2015.01.004, 2015.
Townsend-Small, A., Noguera, J. L., McClain, M. E., and Brandes, J. A.: Radiocarbon and stable isotope geochemistry of organic matter in the Amazon headwaters, Peruvian Andes, Global Biogeochem. Cy., 21, GB2029, https://doi.org/10.1029/2006GB002835, 2007.
van der Voort, T. S., Blattmann, T. M., Usman, M., Montluçon, D., Loeffler, T., Tavagna, M. L., Gruber, N., and Eglinton, T. I.: MOSAIC (Modern Ocean Sediment Archive and Inventory of Carbon): a (radio)carbon-centric database for seafloor surficial sediments, Earth Syst. Sci. Data, 13, 2135–2146, https://doi.org/10.5194/essd-13-2135-2021, 2021.
van Hoek, W. J., Wang, J., Vilmin, L., Beusen, A. H. W., Mogollón, J. M., Müller, G., Pika, P. A., Liu, X., Langeveld, J. J., Bouwman, A. F., and Middelburg, J. J.: Exploring Spatially Explicit Changes in Carbon Budgets of Global River Basins during the 20th Century, Environ. Sci. Technol., 55, 16757–16769, https://doi.org/10.1021/acs.est.1c04605, 2021.
Vonk, J. E., Tank, S. E., Bowden, W. B., Laurion, I., Vincent, W. F., Alekseychik, P., Amyot, M., Billet, M. F., Canário, J., Cory, R. M., Deshpande, B. N., Helbig, M., Jammet, M., Karlsson, J., Larouche, J., MacMillan, G., Rautio, M., Walter Anthony, K. M., and Wickland, K. P.: Reviews and syntheses: Effects of permafrost thaw on Arctic aquatic ecosystems, Biogeosciences, 12, 7129–7167, https://doi.org/10.5194/bg-12-7129-2015, 2015.
Wacker, L., Bonani, G., Friedrich, M., Hajdas, I., Kromer, B., Němec, M., Ruff, M., Suter, M., Synal, H.-A., and Vockenhuber, C.: MICADAS: routine and high-precision radiocarbon dating, Radiocarbon, 52, 252–262, https://doi.org/10.1017/S0033822200045288, 2010.
Wang, J., Jin, Z., Hilton, R. G., Zhang, F., Densmore, A. L., Li, G., and West, A. J.: Controls on fluvial evacuation of sediment from earthquake-triggered landslides, Geology, 43, 115–118, https://doi.org/10.1130/g36157.1, 2015.
Wang, J., Hilton, R. G., Jin, Z., Zhang, F., Densmore, A. L., Gröcke, D. R., Xu, X., Li, G., and West, A. J.: The isotopic composition and fluxes of particulate organic carbon exported from the eastern margin of the Tibetan Plateau, Geochim. Cosmochim. Acta, 252, 1–15, https://doi.org/10.1016/j.gca.2019.02.031, 2019.
Wang, X., Ma, H., Li, R., Song, Z., and Wu, J.: Seasonal fluxes and source variation of organic carbon transported by two major Chinese Rivers: The Yellow River and Changjiang (Yangtze) River, Global Biogeochem. Cy., 26, GB2025, https://doi.org/10.1029/2011gb004130, 2012.
Wild, B., Andersson, A., Bröder, L., Vonk, J., Hugelius, G., McClelland, J. W., Song, W., Raymond, P. A., and Gustafsson, Ö.: Rivers across the Siberian Arctic unearth the patterns of carbon release from thawing permafrost, P. Natl. Acad. Sci. USA, 116, 10280–10285, https://doi.org/10.1073/pnas.1811797116, 2019.
Wu, Y., Eglinton, T. I., Zhang, J., and Montlucon, D. B.: Spatiotemporal Variation of the Quality, Origin, and Age of Particulate Organic Matter Transported by the Yangtze River (Changjiang), J. Geophys. Res., 123, 2908–2921, https://doi.org/10.1029/2017JG004285, 2018.
Zimov, S. A., Schuur, E. A. G., and Chapin, F. S.: Permafrost and the Global Carbon Budget, Science, 312, 1612–1613, https://doi.org/10.1126/science.1128908, 2006.