the Creative Commons Attribution 4.0 License.
the Creative Commons Attribution 4.0 License.
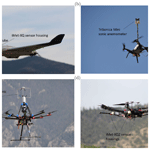
University of Kentucky measurements of wind, temperature, pressure and humidity in support of LAPSE-RATE using multisite fixed-wing and rotorcraft unmanned aerial systems
Michael P. Sama
Caleb A. Canter
L. Felipe Pampolini
Zachary S. Lippay
Travis J. Schuyler
Jonathan D. Hamilton
Sean B. MacPhee
Isaac S. Rowe
Christopher D. Sanders
Virginia G. Smith
Christina N. Vezzi
Harrison M. Wight
Jesse B. Hoagg
Marcelo I. Guzman
Suzanne Weaver Smith
In July 2018, unmanned aerial systems (UASs) were deployed to measure the properties of the lower atmosphere within the San Luis Valley, an elevated valley in Colorado, USA, as part of the Lower Atmospheric Profiling Studies at Elevation – a Remotely-piloted Aircraft Team Experiment (LAPSE-RATE). Measurement objectives included detailing boundary layer transition, canyon cold-air drainage and convection initiation within the valley. Details of the contribution to LAPSE-RATE made by the University of Kentucky are provided here, which include measurements by seven different fixed-wing and rotorcraft UASs totaling over 178 flights with validated data. The data from these coordinated UAS flights consist of thermodynamic and kinematic variables (air temperature, humidity, pressure, wind speed and direction) and include vertical profiles up to 900 m above the ground level and horizontal transects up to 1500 m in length. These measurements have been quality controlled and are openly available in the Zenodo LAPSE-RATE community data repository (https://zenodo.org/communities/lapse-rate/, last access: 23 July 2020), with the University of Kentucky data available at https://doi.org/10.5281/zenodo.3701845 (Bailey et al., 2020).
- Article
(7420 KB) - Full-text XML
- BibTeX
- EndNote
This paper discusses the systems and contribution of the University of Kentucky researchers to the Lower Atmospheric Profiling Studies at Elevation – a Remotely-piloted Aircraft Team Experiment (LAPSE-RATE) campaign (de Boer et al., 2020b) conducted from 13 through 19 July 2018 in the San Luis Valley in Colorado, USA. In this campaign unmanned aerial system (UAS)-based atmospheric science research teams cooperated with researchers from the National Center for Atmospheric Research (NCAR), the National Severe Storms Laboratory (NSSL), and the National Oceanic and Atmospheric Administration (NOAA) (de Boer et al., 2020a) to conduct UAS sensor intercomparison, along with separate days of UAS and ground observations focused on boundary layer transition, canyon cold-air drainage and convection initiation. This paper describes the contribution from University of Kentucky researchers towards the campaign objectives, including a description of the systems used and discussion of the data acquired during the LAPSE-RATE campaign (de Boer et al., 2020b).
University of Kentucky's research in UAS flight testing evolved over more than 450 flights conducted to evaluate performance of deployable-wing unmanned aircraft (Jacob et al., 2005, 2007; Thamann et al., 2015), before expanding into atmospheric turbulence (Witte et al., 2016, 2017), formation and flight control (Mullen et al., 2016; Heintz et al., 2019; Wellman and Hoagg, 2018; Heintz and Hoagg, 2019; Lippay and Hoagg, 2019), and measurement of atmospheric gas concentrations (Schuyler and Guzman, 2017; Schuyler et al., 2019a, b) as well as participants in the Collaboration Leading Operational UAS Development for Meteorology and Atmospheric Physics (CLOUDMAP) program (Jacob et al., 2018). The CLOUDMAP program focused on the development of UAS technologies for meteorology and atmospheric science and resulted in advancement of capabilities for atmospheric observations with UASs, as demonstrated via multiuniversity flight campaigns (Smith et al., 2017), via validation experiments (Barbieri et al., 2019) and through observations of the surface layer transitions during the 2017 total eclipse (Bailey et al., 2019).
For the LAPSE-RATE campaign, the University of Kentucky deployed four fixed-wing UASs and three rotorcraft UASs, which measured pressure, temperature, relative humidity, horizontal wind magnitude, vertical wind magnitude and direction. The UASs were used to measure these thermodynamic and kinematic variables at altitudes up to 900 m above ground level (a.g.l.). A surface flux tower was also deployed, capable of measuring net solar radiation, soil temperature, ground heat flux, surface wind and turbulence and the near-surface temperature gradient. These assets were deployed to seven different sites over the course of LAPSE-RATE, measuring at up to five sites simultaneously to contribute to scientific objectives targeting convection initiation, boundary layer transition and cold-air drainage. Over the course of the week, the team conducted over 178 flights yielding vetted observation data. Details about the systems used, their deployment, and quality-control checks applied to the data are described in the remainder of the paper, with the data files openly available (Bailey et al., 2020).
The following sections of this paper include descriptions of the diverse UASs and ground systems used by the University of Kentucky during LAPSE-RATE, followed by details of measurement locations and completed flights. Data processing and quality-control specifics are included, which provide information needed by those wanting to use these data. Examples of some of the features observed within the data are highlighted as well.
2.1 BLUECAT5 UAS
Four fixed-wing UASs were used by the University of Kentucky during the LAPSE-RATE measurement campaign. These UASs, of which one is shown in Fig. 1a, were constructed from Skywalker X8 airframes, modified to introduce semiautonomous operation by integrating a 3DR Pixhawk autopilot, and ruggedized by strengthening wing spars, skinning the aircraft, adding Kevlar landing skids, and shielding to minimize the ingestion of dirt into the motor. Referred to as the Boundary Layer Unmanned Experiment for the Characterization of Atmospheric Turbulence generation five (BLUECAT5) UASs (Witte et al., 2017), the aircraft had endurance of up to 45 min with 20 m s−1 cruise speeds and were catapult launched and skid landed. The four aircraft used here are identified as BCT5B, BCT5C, BCT5D and BCT5E.
In the configuration used for LAPSE-RATE, the instrumentation allowed for the measurement of three components of wind, as well as pressure, temperature and humidity. Airspeed information used by the autopilot was acquired using a 30 cm long pitot–static tube extending from the nose of the aircraft. In addition, the pitot–static tube was used to provide a reference static pressure for a custom-manufactured five-hole pressure probe used to measure the velocity vector of the air relative to the aircraft. Pressure readings from each port of the five-hole probe were acquired using TE Connectivity, Switzerland, 4515-DS5A002DP differential pressure transducers with a 0.5 kPa range. Analog output from the sensors was digitized at 16 bit resolution at a rate of 400 Hz with a 200 Hz passive resistor–capacitor low-pass filter used for anti-aliasing. Digitization was performed using a MCC-DAQ USB-1608FS-PLUS multifunction data acquisition system controlled by a Kangaroo portable PC.
Each five-hole probe was calibrated for directional response using a 0.6 m×0.6 m wind tunnel. The calibration followed a standard calibration technique outlined by Treaster and Yocum (1978) which was implemented following the results presented by Wildmann et al. (2014). During these studies, the probe tubing length was optimized to provide a frequency response on the order of 100 Hz. At the typical cruise speed of BLUECAT5, this frequency response translates to a spatial measurement resolution of approximately 0.2 m. Additional wind and water tunnel studies verified that positioning the measurement volume of the probe 18 cm upstream of the nose of the aircraft was sufficient to minimize interference effects from the airframe (Witte et al., 2017).
Six-degrees-of-freedom position and rate information was provided by a VN-300 manufactured by VectorNav. The VN-300 provided a heading accuracy of and pitch/roll accuracy of with ground velocity accuracy of ±0.05 m s−1. The orientation information was sampled at 200 Hz by custom software running on the Kangaroo PC.
Pressure, temperature and humidity were measured using an International Met Systems iMet-XQ UAV sensor. The iMet pressure sensor provides a ±1.5 hPa accuracy for pressure, with the humidity sensor supporting a full 0–100 % RH range at ± 5 % RH accuracy with a resolution of 0.7 % RH. The iMet-XQ temperature sensor provides a ±0.3 ∘C accuracy with a resolution of 0.01 ∘C up to a maximum of 50 ∘C. The stated response times of these sensors are on the order of 10 ms for pressure, 5 s for humidity and 2 s for temperature in still air, with the iMet-XQ UAV system sampling these sensors at 1 Hz. The iMet-XQ sensor was mounted on top of the aircraft with the thermistor exposed to the airflow but shielded by a 3D-printed acrylonitrile butadiene styrene arch designed to protect it from heating via solar radiation.
To determine the wind velocity vector, the velocity of the air measured by the five-hole probe in an aircraft-fixed frame of reference is transformed into an Earth-fixed frame of reference using inertial velocity provided by the VN-300. Following this transformation, the aircraft's velocity relative to the ground was subtracted, leaving the three-component wind vector (Axford, 1968; Lenschow, 1972; Broxmeyer and Leondes, 1964). Further details are available in Witte et al. (2017) with additional corrections applied as described in Al-Ghussain and Bailey (2020) to correct for probe orientation bias. During this postprocessing step, all data were resampled to 200 Hz and aligned in time via cross correlation of the signals measured by the different systems. However, the data from these aircraft should only be considered to be resolved to 100 Hz for wind, 1 Hz for pressure, 0.5 Hz for temperature and 0.2 Hz for humidity.
2.2 SOLOW UAS
Pressure, temperature, humidity and wind measurements were also conducted using a 3DRobotics Solo quadrotor identified here as the SOLOW and shown in Fig. 1b. This UAS was capable of semiautonomous flight through a Pixhawk autopilot and had approximately 10 min of endurance for each battery, but near-continuous operations were possible by keeping the sufficient batteries charged and available to ensure that the aircraft could be returned to flight with approximately 3 to 5 min on the ground for a battery change. Used for vertical profiling measurements, this aircraft was typically operated with 2 m s−1 descent and 3 m s−1 ascent velocities, which optimizes the number of vertical profiles which could be obtained from a single battery charge while maintaining the thermodynamic data within a nominally 2.5 m measurement resolution.
For measuring the pressure, temperature and relative humidity, the UAS was equipped with an International Met Systems iMet-XQ-2 UAV sensor, in a custom mount below one of the rotors to ensure sufficient aspiration of the sensors with solar radiation shielding of the humidity and thermistor provided by the mount. Wind speed and direction sensing was provided by a TriSonica Mini sonic anemometer manufactured by Applied Technologies with a manufacturer-provided accuracy of ±0.1 m s−1 and in wind speed and direction respectively. The anemometer was mounted on a 0.38 m carbon fiber post above the main body of the rotorcraft with the optimal mast height determined through laboratory tests consisting of increasing the post length until the UAS motors running at full speed did not result in a change in anemometer reading. Additional laboratory calibration was conducted in a 0.6 m×0.6 m wind tunnel to account for blockage effects from the sensor housing with calibration applied a posteriori. Although nominally a three-component anemometer, it had an acceptance cone for vertical winds of to the vertical which, when exceeded, contaminated the horizontal components of velocity as discussed in Sect. 4.
Digital output from the sonic anemometer was logged at 10 Hz during flight using a Slerj RS232 data logger and analyzed a posteriori following the same procedures utilized for the fixed-wing aircraft, but with the aircraft position and orientation information extracted at 10 Hz from the autopilot flight logs. Corresponding pressure, temperature and humidity data were interpolated to 10 Hz but should only be considered resolved to 1 Hz for pressure, 0.5 Hz for temperature and 0.2 Hz for humidity.
2.3 S1000 UAS
Pressure, temperature, humidity and wind measurements were also conducted using a DJI S1000 octocopter, identified here as the S1000, shown in Fig. 1c. The S1000 was modified for semiautonomous operation by implementing a Pixhawk autopilot and was capable of approximately 20 min of flight time for each battery pack. Used for vertical profiling measurements, this aircraft was typically operated with 1 m s−1 descent and ascent velocities, which optimizes the altitude which could be obtained for flight measuring a single vertical profile on a single battery charge while minimizing the spatial resolution of the thermodynamic data to nominally 1 m.
Pressure temperature and relative humidity measurements were provided by an International Met Systems iMet-XQ system. These systems log data at 1 Hz, with a stated response and accuracy of 10 ms, ±1.5 hPa for pressure; 1 s, ±0.3 ∘C for temperature; and 0.6 s, 4±0.5 % RH for relative humidity. The sensors were located under one of the aircraft's rotors for sensor aspiration in a housing designed to minimize the impact of solar radiation on the sensing.
To measure wind speed and direction an R.M. Young, USA, model 81000 ultrasonic anemometer was mounted on a mast with its measurement volume located 0.55 m above the rotor plane, at the centerline of the aircraft. The Young 81000 could measure wind speeds up to 40 m s−1 at a resolution of 0.01 m s−1 with an accuracy of ±0.05 m s−1. The anemometer was set to output velocity and sonic temperature data as analog signals, which were digitized at a nominal rate of 70 Hz by a Mayhew labs 16 bit analog-to-digital converter controlled by an Arduino embedded computer.
As with the SOLOW UAS, the data were processed a posteriori to subtract aircraft motion from the wind data. To do so the aircraft position and orientation information, sonic anemometer data and iMet-XQ data were interpolated to 10 Hz. Although pressure, temperature and humidity data were interpolated to 10 Hz, they should only be considered resolved to 1 Hz for this aircraft.
2.4 M600P UAS
An additional UAS was used for pressure, temperature and humidity profiling, based on the DJI M600P hexacopter platform. This aircraft, shown in Fig. 1d, used the manufacturer-provided batteries and autopilot, allowing for semiautonomous operation with approximately 20 min of flight time. Here we identify this UAS as the M600P.
Instrumentation on this aircraft was provided by two International Met iMet-XQ2 sensing systems. These sensors are the same type as described above in the description of the S1000; however, on the M600P these two were mounted below opposite rotors to maintain weight and balance. Data from both sensors were logged independently at 1 Hz.
Unlike with the other UASs, the autopilot data were not available for use in postprocessing the iMet-XQ2 sensor data. Instead, the intrinsic iMet-XQ2 GPS and altimeter data were used to identify UAS position and altitude information, with the pressure, temperature and humidity data from one sensor interpolated to sample times corresponding to the other sensor to unify the data stream. The intrinsic iMet-XQ2 GPS was a u-blox CAM-M8 with a vertical accuracy of 12 m and response time of 1 s.
2.5 Flux tower
Additional ground-based measurements were provided by a 2 m surface flux tower shown in Fig. 2. The tower was equipped with a three-component sonic anemometer (Campbell Scientific CSAT-3) for measuring wind speed and direction. Sensor accuracy was between ±2 % and ±6 % with ±0.08 m s−1 bias precision. Output from the anemometer was logged at 20 Hz via RS232 using a Kangaroo portable computer mounted in a weatherproof enclosure.
The tower was equipped with a Campbell Scientific E+E Elektronik EE181 digital temperature and humidity sensor at 2 m having accuracy of ±0.2 ∘C and ±2.3 % RH. At 1.5 and 0.75 m the tower had two additional Campbell Scientific CS215 digital temperature and humidity sensors (±0.4 ∘C, ±4 % RH). All temperature and humidity sensors were housed in a solar radiation shield and logged every 3 s via a Campbell Scientific CR1000X measurement and control data logger.
Additional sensors on the flux tower logged every 3 s included a Setra 278 digital barometer, a Kipp & Zonen NR-LITE2 net radiometer, two Hukseflux HFP01 soil heat flux plates, a Campbell Scientific CS655 water content reflectometer, and a Campbell Scientific TCAV averaging soil thermocouple probe. All sensors were factory calibrated within 1 year of use, although intercomparison measurements in a laboratory environment revealed that the EE181 sensor had a consistent 0.5 ∘C bias which was removed from the measurements reported here.
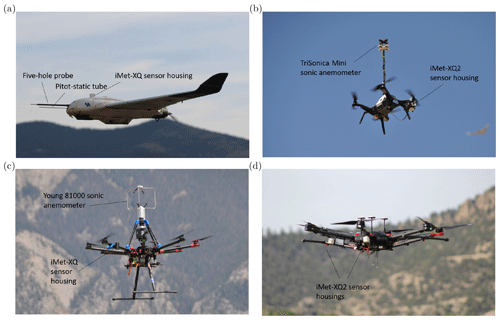
Figure 1Photographs of (a) BCT5, (b) SOLOW, (c) S1000, and (d) M600P showing location of sensors on each aircraft.
The systems described above were deployed at different locations throughout the LAPSE-RATE measurement campaign. These locations are identified as Leach Airfield, Echo, Foxtrot, Kilo, Oscar, Saguache Airfield and Poison Gulch. Approximate latitude and longitude of these locations are provided in Table 1, with flight locations graphically illustrated in Fig. 3. Flight locations and UAS dispositions varied by day of operations and measurement objective, with UAS measurements being conducted in the morning and early afternoon. Aircraft dispositions and measurement times are outlined below and summarized in Table 2. The flux tower was located at Leach Airfield and was measuring continuously until the afternoon of 18 July 2018.
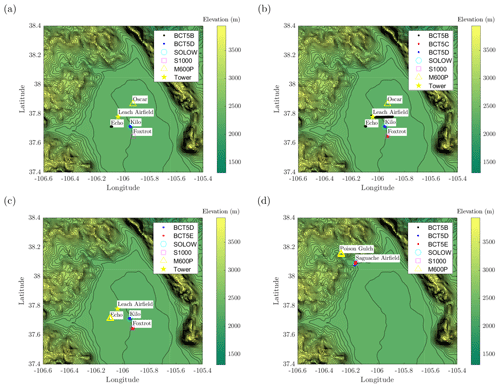
Figure 3Topographic map of northern half of San Luis Valley, Colorado, USA, showing location of tower and aircraft flights conducted by BCT5, S1000, SOLOW, and M600P aircraft on (a) 15 July 2018, (b) 16 July 2018, (c) 18 July 2018 and (d) 19 July 2018. Contour lines indicate a 50 m change in elevation.
The Leach Airfield, Echo, Foxtrot, Kilo and Oscar sites were centrally located in the San Luis Valley, consisting largely of agricultural land having minimal elevation change and loose, dry soil. The Poison Gulch and Saguache Airfield sites were located at two points along a narrow valley leading into the larger San Luis Valley. The Poison Gulch site was a location where the valley was narrow with steep valley walls, whereas the Saguache Airfield site was located closer to the mouth of the valley, where the valley was much broader and had shallower elevation changes.
Civil twilight during the measurement period started between 05:24 MDT on 15 July and 05:27 MDT on 19 July, with the corresponding sunrise times being between 05:54 and 05:57 MDT. Sunsets for the same period were between 20:26 and 20:24 MDT, with the civil twilight ending between 20:57 and 20:54 MDT.
Table 1Latitude and longitude of operation locations in World Geodetic System 84 (WGS 84) decimal degrees.
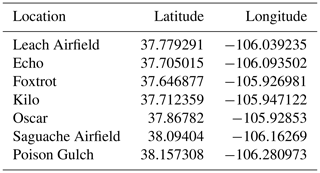
Table 2Measurement systems location. (P) indicates measurement of a vertical profile; (T) indicates a horizontal transect measurement.
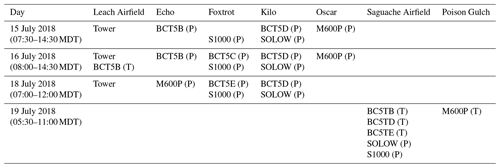
All flights were conducted legally under either FAA Part 107 regulations, which limited flight operations to 121 m or below, or under the University of Kentucky's Federal Aviation Authority (FAA) Certificate of Authority (COA) 2018-WSA-1730-COA effective from 13 to 22 July 2018. The COA authorized operation of small UAS weighing less than 55 pounds (25 kg) and operating at speeds of less than 87 knots (45 m s−1) in Class E and G airspace below 914 m a.g.l. and not exceeding 3657 m above mean sea level (m.s.l.) in the vicinity of Alamosa County, Colorado, under the jurisdiction of the Denver Air Route Traffic Control Center (ARTCC). Standard COA provisions were applied, including those for airworthiness, operations, flight crew, safety, notices to airmen (NOTAMs), reporting and registration. Special provisions were also necessary for coordination and deconfliction of operations of multiple organizations. Rather than authorizing different overlapping operating areas defined by the preplanned positioning for each organization, discussions among the organizations, FAA and Denver air traffic control, led to definition of one common area encompassing the entire LAPSE-RATE plan of operations. With this, additional coordination and deconfliction became necessary with airports and several intersecting military training routes (MTRs). Two NOTAM subareas were defined so that one or both could be issued with the required 24 h notice depending on the selected weather question that would be pursued the next day. Emergency contingency procedures for lost links, communications and other potential anomalies also had special provisions due to the proximity of operations of the various organizations.
3.1 15 July 2018
Measurements investigating convection initiation were scheduled for 15 July 2018. To support this objective, vertical profiling flights measuring kinematic and thermodynamic parameters were conducted at Echo, Foxtrot, Kilo and Oscar, with BC5TB at Echo, the S1000 at Foxtrot, BCT5D and the SOLOW at Kilo, and the M600P at Oscar. The nominal flight profile for these flights was for the fixed-wing UAS to conduct 5 m s−1, 200 m diameter spiraling ascents and descents to 900 m a.g.l., with the rotorcraft ascending and descending vertically to 300 m a.g.l. The S1000 measured a single profile per flight ascending/descending at 1 m s−1, whereas the SOLOW completed two ascents/descents at 3 m s−1 upward and 2 m s−1 downward velocity. The UAS repeated these patterns from takeoff until their battery life was expended, at which point they would land and have their batteries changed. The nominal tempo for these patterns was to conduct these flights once per hour. At Kilo, where two aircraft were available, the flights were staggered such that one aircraft was launched every 30 min. A figure showing the height, z, of each aircraft as a function in time is presented in Fig. 4a. This figure serves as a graphical representation of the time each aircraft flew over the course of the day.
On this day, the wind speed and direction was not measured by the SOLOW aircraft due to data acquisition issue which was not diagnosed until after flights had concluded. In addition, the S1000 conducted limited profiles at Foxtrot due to overheating of the flight batteries.
3.2 16 July 2018
Measurements investigating convection initiation were also scheduled for 16 July 2018, and flight operations were expected to be a repeat of 15 July 2018, with vertical profiling flights measuring kinematic and thermodynamic parameters. UASs were located at Echo, Foxtrot, Kilo and Oscar, with BC5TB at Echo, BCT5C and the S1000 at Foxtrot, BCT5D and the SOLOW at Kilo, and the M600P at Oscar. However, it was discovered that the NOTAM was not active for any operation locations (excepting Oscar), so flight operations could not be conducted under the COA regulations, requiring flights to be restricted to 120 m and below. As a result, it was decided that the fixed-wing operations could be better utilized, and their flight crews were retasked to conduct horizontal east–west transects with BCT5B to attempt to capture any fronts descending from the Sangre de Cristo Mountains to the east. Two 13 km long transects were conducted along the path initiating at Leach Airport (see Fig. 3b) in conjunction with a ground vehicle from the University of Nebraska–Lincoln.
To compensate for BC5TD being retasked, the cadence of the SOLOW operations were increased to nominally one 1 m s−1 ascent/descent profile measurement to 120 m every 15 min. However, due to continued battery issues, the S1000 was not able to maintain this cadence, and separation between 1 m s−1 ascent/descent profile measurements to 120 m was between 30 and 60 min. A graphical representation of the time each aircraft flew over the course of the day is presented in Fig. 4b. Note that some discrepancies exist in the M600P profiles exist caused by poor GPS performance from the onboard systems on this day.
3.3 18 July 2018
Measurements investigating boundary layer transition were scheduled for 18 July 2018. As a result, profiling flights were again conducted following the same flight patterns as on 15 July. However, due to concerns with potential low-altitude manned aircraft flights through the VR-413 airway, the M600P was retasked to Echo. A fixed-wing and rotorcraft UASs were located at Foxtrot and Kilo, with BCT5E and the S1000 at Foxtrot, and BCT5D and the SOLOW at Kilo. Flight operations on this day largely went as scheduled, with the cadence presented in Fig. 4c.
3.4 19 July 2018
Measurements of the cold-air drainage into the valley were scheduled for 19 July 2018. For this study, all aircraft were operating in the narrow valley leading into Saguache, with the flight locations and patterns indicated in Fig. 5a for Poison Gulch and Fig. 5b for Saguache Airfield. Most aircraft were operating at Saguache Airfield, with the M600P operating further up the mouth of the valley, in Poison Gulch.
At Poison Gulch, the M600P was flying 600 m long horizontal transects at 25 m a.g.l. and every 50 m up to 250 m a.g.l. at 10 m s−1 every 30 min to capture the flow entering the mouth of the valley. To measure the flow approaching the mouth of the valley, the remainder of the aircraft were positioned at Saguache Airfield. To capture the horizontal distribution of the thermodynamic and kinematic variables, the fixed-wing aircraft measured nominally 1700 m long transects at four different altitudes with the rotorcraft flying vertical profiles. The SOLOW rotorcraft was conducting near-continuous profiles at 2 to 3 m s−1 up to 100 m from 05:30 to 11:00 MDT, with the S1000 profiling at 1 m s−1 every hour from 06:00 to 11:00 MDT up to 300 m. The transects were measured at a nominal altitude of 100, 150, and 200 and 400 m a.g.l. Due to a longer preparation time for the fixed-wing aircraft, full operations did not begin until 07:00 MDT, with flights continuing to 10:30 MDT. The actual flight times and altitudes for all aircraft are presented in Fig. 4d.
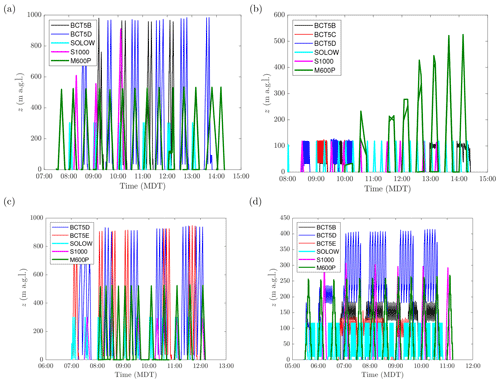
Figure 4Altitude above ground level of each aircraft as a function of time for (a) 15 July 2018, (b) 16 July 2018, (c) 18 July 2018 and (d) 19 July 2018.
Inspection of the raw data from these flights revealed several instances were the instrumentation was not functioning properly. As noted above, the SOLOW sonic anemometer was not powered during the 15 July measurements, and no wind data were recovered from those flights. On 17 July, the pressure, temperature and humidity from the SOLOW only seem to have been recorded in the first two flights. In addition, a power issue was also discovered with the S1000 anemometer, whereby the anemometer would shut down approximately halfway through the profile. This problem persisted throughout the campaign. Finally, several fixed-wing flights encountered overheating and malfunction of the embedded computer, resulting in a loss of wind data for these flights. Where these faults were observed, the values in the files have been replaced with values of −9999.9.
Table 3Overview of measurement systems and associated uncertainties. Measured quantities are pressure (P ), temperature (T), relative humidity (RH), wind velocity components in the horizontal and vertical direction respectively (u,v and w), net solar radiation (Net IR), and volumetric water content (VWC).
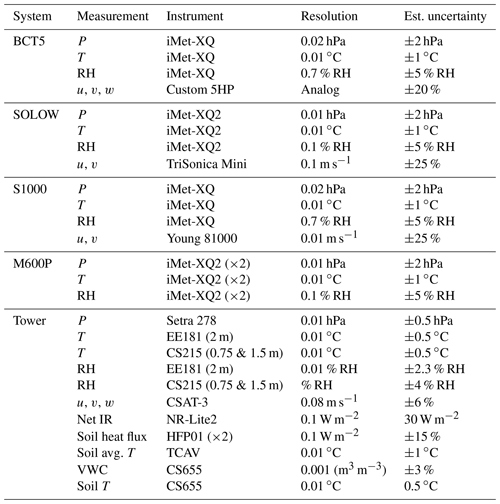
Furthermore, erroneous readings were observed for the SOLOW anemometer during profiling flights which were caused by vertical acceptance angle violations. Wind data from this system should only be considered to be reliable when the aircraft was descending due to a lower vertical velocity during descent. These instances have been replaced in the data files with −9999.9. In addition, slight misalignment between ground and relative air velocity measurements introduces large biases in the wind estimates provided by the BCT5 aircraft during periods of significant acceleration, specifically during takeoff, landing and sharp turns. These biases were removed using the approach described in Al-Ghussain and Bailey (2020). However, wind data from the time periods when these aircraft are entering or leaving their measurement profile should be considered unreliable due to accelerations which exceeded the ability of the approach to detect and remove.
As noted in the individual system descriptions, several laboratory calibrations were conducted prior to the flight campaign, most notably for the systems for measuring wind. In addition, as part of an intercomparison study, a series of flights were conducted on 14 July 2018 near a ground-based reference tower. The results of this study, published in Barbieri et al. (2019), indicated an overestimation of the winds measured by the BCT5 aircraft by up to 10 % to 20 %. Additional laboratory calibrations of the S1000 anemometer indicated that the horizontal winds were underestimated by 25 %.
These biases were found to be constant throughout the campaign and independent of weather conditions and flight profile, as intrinsically validated by intercomparison between all systems and the Automated Surface Observing System (ASOS) using the colocated flights at Saguache Airfield. As the wind direction was found to be unaffected, they have therefore been uniformly removed from the data files by applying them equally to both horizontal components of velocity. However the magnitude of these corrections do reflect the level of uncertainty in the respective wind measurements. In addition, utilizing the Barbieri et al. (2019) intercomparison study findings, we also find that the temperature has an absolute confidence level of ±1 ∘C, the relative humidity values have an absolute confidence level of approximately ±5 % and the pressure values have an absolute confidence level of ±2 hPa. An overview of the systems, their instruments, and corresponding resolution and estimated uncertainty is provided in Table 3.
This section provides a general overview of the data provided by the systems described in Sect. 2 during the LAPSE-RATE campaign from the mornings of 15, 16, 18 and 19 July. An overview of the prevailing synoptic and mesoscale conditions during the week is provided in de Boer et al. (2020b), as well as de Boer et al. (2020a).
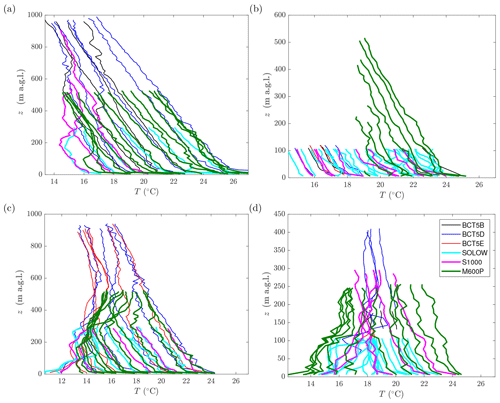
Figure 7Temperature measured by aircraft on (a) 15 July 2018, (b) 16 July 2018, (c) 18 July 2018 and (d) 19 July 2018. Note different axis heights for each day. Profiles have been produced by averaging over 10 m height intervals from each flight.
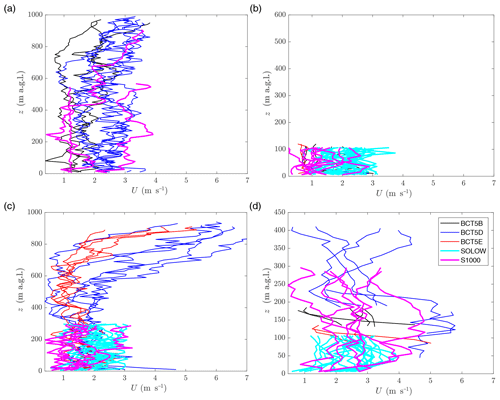
Figure 8Wind velocity magnitude: (a) 15 July 2018, (b) 16 July 2018, (c) 18 July 2018 and (d) 19 July 2018. Axis heights same as Fig. 7. Profiles have been produced by averaging over 10 m height intervals from each flight.
The measurements made by the 2 m flux tower are presented in Fig. 6. Figure 6a shows that surface winds from 15 to 18 July were variable in direction, generally below 5 m s−1 but picking up in the late afternoon with the development of convective boundary layer conditions, occasionally reaching velocity magnitudes exceeding 10 m s−1. Convective thunderstorm activity occurred in the afternoons as well, resulting in additional wind gusts corresponding to their outflows. A consistent diurnal cycle was observed for 15 through 18 July in heat flux (Fig. 6b); temperature, T (Fig. 6c); relative humidity, RH (Fig. 6d); and volumetric water content, VWC (Fig. 6e). The calm winds in the mornings corresponded to elevated humidity, with the 100 % RH observed on the morning of 18 July corresponding to early-morning fog formation. Near-surface air temperatures ranged from 10 to 30 ∘C with soil temperatures following the same cycle but elevated to between 20 and 50 ∘C. The volumetric water content was low, reflecting the dry soil conditions prevalent in the San Luis Valley (evident in Fig. 2).
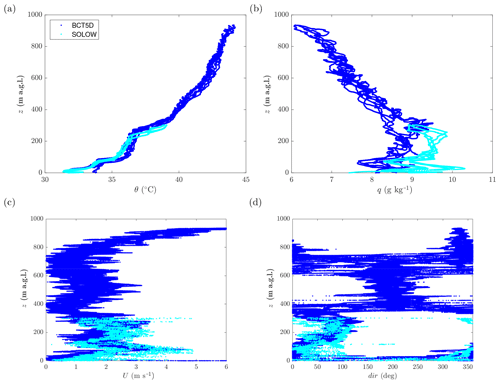
Figure 9Example vertical profiles measured between 07:00 and 07:45 MDT by BCT5D and SOLOW on 18 July 2018 at location Kilo. Potential temperature profiles shown in (a); water vapor mass mixing ratio profiles shown in (b); and wind speed, u, and direction, dir, profiles shown in (c) and (d) respectively.
Mean vertical profiles from all temperature measurements made are presented in Fig. 7 with the corresponding velocity magnitude profiles presented in Fig. 8. These profiles were produced by bin averaging the results at 10 m intervals for each flight. The temperature profiles from 15 July, which were measured between 07:30 and 14:30 MDT and are presented in Fig. 7a, show typical transition behavior, with the lower-temperature, early-morning profiles showing a nonmonotonic altitude dependence up to 800 m a.g.l., whereas the later afternoon profiles consist of the characteristic lapse rate of a well-mixed convective boundary layer. Over the same time period, the wind profiles shown in Fig. 8a indicate the presence of calm winds of generally less than 3 m s−1 magnitude. Wind profiles were essentially the same on 16 July between 08:00 and 14:30 MDT, shown in Fig. 8b, although the altitude restrictions described in Sect. 3.2 limited measurements of winds to the lowest 100 m. Likewise, the temperature profiles shown in Fig. 7b show a consistent lapse rate throughout the morning. More interesting boundary layer transition behavior was evident on 18 July between 07:00 and 12:00 MDT. The temperature profiles shown in Fig. 7c indicate the presence of multiple inversions during the measurements made earlier in the morning, which evolve into a well-mixed lapse rate as surface temperatures increase. The corresponding wind profiles shown in Fig. 8c show that, although relatively calm winds were evident near the surface during the entire measurement period, significantly stronger winds and wind shear existed above 500 m a.g.l. Finally, the measurements made in the Saguache valley on 19 July between 05:30 and 11:00 MDT show in Fig. 7d the expected reversal of gradient in the temperature profiles associated with the earlier morning boundary layer behavior as it transitions to mixed-layer conditions over the course of the morning. As expected over the complex terrain of the valley, the winds were variable as shown in Fig. 8d, with mean velocity peak near 6 m s−1 caused by a transient density-driven flow event.
Of the 178 measurements made, as noted in the previous section some of the most interesting profiles were measured on the morning of 18 July 2018. On this morning the weather was fair, having less than three-eights cloud cover, with surface winds varying from calm to 2.5 m s−1. Surface temperature increased from 10 to 25 ∘C, with the dew point dropping from 5 to −1 ∘C throughout the morning. However the boundary layer was stratified into multiple layers evident in each of the measured statistics, as illustrated in the sample profiles from some of the earliest colocated flights on that day which are presented in Fig. 9. In Fig. 9, profiles from the SOLOW rotorcraft are shown for a 10 min flight initiating at 07:00 MDT and profiles from BCT5D are shown for a flight initiating at 07:15 MDT that lasted 30 min. Hence the time period represented by these profiles is approximately 45 min and any features evident persisted over that duration. Note that, despite this time difference, no dependence of measured values on ascent or descent can be observed in these measurements, and there appears to be good agreement between the different aircraft and sensor systems.
The potential temperature profiles, shown in Fig. 9a, suggests that the boundary layer was largely stable, except for a thin region of unstable air near the surface. Significant changes in the potential temperature, θ, gradient are evident at 50, 100, 250 and 300 m a.g.l. The corresponding water vapor mass mixing ratio, q, profiles, shown in Fig. 9b, are slightly less complex, but changes in the vertical gradient are also observed at 50, 100 and 250 m a.g.l., coinciding with the changes observed in the potential temperature. The wind horizontal magnitude and direction are shown in Fig. 9c and d respectively. These figures show that the winds also experienced significant vertical and horizontal shearing. For the lowest 100 m a.g.l., winds were from the north at up to 3 m s−1, whereas they were from the east at 2 m s−1 from 100 to 300 m a.g.l. From 300 to 600 m a.g.l. they were from the south at 2 m s−1, and above that altitude the winds were from the north, increasing in magnitude with altitude until reaching 6 m s−1 at 900 m. Notably, these trends were evident on both aircraft systems at altitudes where overlapping measurements are available. Similar trends were observed for other UASs throughout the morning during the same period, with mixed-layer conditions establishing up to 300 m a.g.l. at 09:15 MDT, up to 500 m a.g.l. at 10:15 MDT, 600 m a.g.l. at 11:15 MDT and throughout the measurement domain at 12:00 MDT.
The data files for each flight from each aircraft are available from the Zenodo open data repository (https://doi.org/10.5281/zenodo.3701845, Bailey et al., 2020). These data consist of 178 files containing thermodynamic and kinematic data (pressure, temperature, humidity, wind speed and direction) measured by the unmanned aerial systems and fixed 2 m flux tower operated by the University of Kentucky during the LAPSE-RATE campaign. These data have undergone preliminary quality control in the form of bias corrections and elimination of some false readings. Files are posted for each individual UAS flight in netCDF format. For the flux tower data, each measurement day is presented as a separate netCDF file.
The netCDF files have each variable listed individually with self-describing metadata to provide information on the source and units for the data. Missing data points or those determined to have bad values have been set to −9999.9.
Files are named using the following standard: UKY.ppppp.b1.yyyymmdd.hhmmss.cdf, with ppppp being the five-letter platform identifier, yyyymmdd being the file date (UTC year, month, day, month) and hhmmss being the file start time (UTC hours, minutes, seconds).
In July 2018 in the San Luis Valley in Colorado, USA, researchers from multiple institutions participated in the Lower Atmospheric Profiling Studies at Elevation – a Remotely-piloted Aircraft Team Experiment (LAPSE-RATE) measurement campaign. As part of this campaign effort, University of Kentucky researchers contributed seven fixed-wing and rotorcraft unmanned aerial systems, totaling 178 successful data acquisition flights over 4 d. For 3 of the 4 d, the flights consisted of profiles measured up to altitudes of 900 m above ground level. The goal of these flights was to observe the boundary layer state during morning transition within the elevated valley and to identify precursors for convection initiation. For the fourth day the gravity-driven flow into the valley was measured using three fixed-wing-aircraft-conducted transects and one rotorcraft-aircraft-conducted transects at six altitudes while the remaining aircraft conducted vertical profiling.
The data from these coordinated UAS flights provide a significant contribution to the characterization of the lower atmosphere within the valley during the LAPSE-RATE campaign. All data from the systems described here are now distributed and can be freely accessed from the Zenodo data repository (https://doi.org/10.5281/zenodo.3701845, Bailey et al., 2020). Although some preliminary quality control and bias correction have been conducted on these data, some caveats for their use have been described in this paper. These caveats include noting that data from the BC5T and SOLOW aircraft are sampled at rates exceeding that of the response for their respective sensing systems.
The University of Kentucky contribution to LAPSE-RATE was planned and coordinated by SWS, MPS, JBH, MIG and SCCB; flight team members included CAC, LFP, ZSL, TJS, JDH, SBM, ISR, CDS, VGS, CNV and HMW; data analysis and preparation was conducted by SCCB; and senior team members SCCB, SWS and MPS contributed to the preparation of this paper.
The authors declare that they have no conflict of interest.
This article is part of the special issue “Observational and model data from the 2018 Lower Atmospheric Process Studies at Elevation – a Remotely-piloted Aircraft Team Experiment (LAPSE-RATE) campaign”. It is a result of the International Society for Atmospheric Research using Remotely piloted Aircraft (ISARRA 2018) conference, Boulder, USA, 9–12 July 2018.
This research has been supported by the National Science Foundation, Division of Chemical, Bioengineering, Environmental, and Transport Systems (grant no. CBET-1351411), and the National Science Foundation, Office of Experimental Program to Stimulate Competitive Research (grant no. 1539070). Additional student financial travel support was provided through the National Science Foundation (grant no. AGS-1807199) and Department of Energy (grant no. DE-SC0018985).
This paper was edited by Gijs de Boer and reviewed by two anonymous referees.
Al-Ghussain, L. and Bailey, S. C. C.: An Approach to Minimize Aircraft Motion Bias in Multi-Hole Probe Wind Measurements made by Small Unmanned Aerial Systems, Atmos. Meas. Tech. Discuss., https://doi.org/10.5194/amt-2020-126, in review, 2020. a, b
Axford, D. N.: On the Accuracy of Wind Measurements Using an Inertial Platform in an Aircraft, and an Example of a Measurement of the Vertical Mesostructure of the Atmosphere, J. Appl. Meteorol., 7, 645–666, https://doi.org/10.1175/1520-0450(1968)007<0645:OTAOWM>2.0.CO;2, 1968. a
Bailey, S. C. C., Canter, C. A., Sama, M. P., Houston, A. L., and Smith, S. W.: Unmanned aerial vehicles reveal the impact of a total solar eclipse on the atmospheric surface layer, P. Roy. Soc. A, 475, 20190212, https://doi.org/10.1098/rspa.2019.0212, 2019. a
Bailey, S. C. C., Smith, S. W., and Sama, M. P.: University of Kentucky files from LAPSE-RATE, Zenodo, https://doi.org/10.5281/zenodo.3701845, 2020. a, b, c, d
Barbieri, L., Kral, S. T., Bailey, S. C. C., Frazier, A. E., Jacob, J. D., Reuder, J., Brus, D., Chilson, P. B., Crick, C., Detweiler, C., Doddi, A., Elston, J., Foroutan, H., González-Rocha, J., Greene, B. R., Guzman, M. I., Houston, A. L., Islam, A., Kemppinen, O., Lawrence, D., Pillar-Little, E. A., Ross, S. D., Sama, M. P., Schmale, D. G., Schuyler, T. J., Shankar, A., Smith, S. W., Waugh, S., Dixon, C., Borenstein, S., and de Boer, G.: Intercomparison of Small Unmanned Aircraft System (sUAS) Measurements for Atmospheric Science during the LAPSE-RATE Campaign, Sensors, 19, 2179, https://doi.org/10.3390/s19092179, 2019. a, b, c
Broxmeyer, C. and Leondes, C. I.: Inertial Navigation Systems, J. Appl. Mech., 31, 735, https://doi.org/10.1115/1.3629763, 1964. a
de Boer, G., Diehl, C., Jacob, J., Houston, A., Smith, S. W., Chilson, P., Schmale, David G., I., Intrieri, J., Pinto, J., Elston, J., Brus, D., Kemppinen, O., Clark, A., Lawrence, D., Bailey, S. C. C., Sama, M. P., Frazier, A., Crick, C., Natalie, V., Pillar-Little, E., Klein, P., Waugh, S., Lundquist, J. K., Barbieri, L., Kral, S. T., Jensen, A. A., Dixon, C., Borenstein, S., Hesselius, D., Human, K., Hall, P., Argrow, B., Thornberry, T., Wright, R., and Kelly, J. T.: Development of Community, Capabilities, and Understanding through Unmanned Aircraft-Based Atmospheric Research: The LAPSE-RATE Campaign, B. Am. Meteorol. Soc., 101, E684–E699, https://doi.org/10.1175/BAMS-D-19-0050.1, 2020a. a, b
de Boer, G., Houston, A., Jacob, J., Chilson, P. B., Smith, S. W., Argrow, B., Lawrence, D., Elston, J., Brus, D., Kemppinen, O., Klein, P., Lundquist, J. K., Waugh, S., Bailey, S. C. C., Frazier, A., Sama, M. P., Crick, C., Schmale III, D., Pinto, J., Pillar-Little, E. A., Natalie, V., and Jensen, A.: Data Generated During the 2018 LAPSE-RATE Campaign: An Introduction and Overview, Earth Syst. Sci. Data Discuss., https://doi.org/10.5194/essd-2020-98, in review, 2020b. a, b, c
Heintz, C. and Hoagg, J. B.: Formation Control in a Leader-Fixed Frame for Agents with Extended Unicycle Dynamics that Include Orientation Kinematics on SO(m), in: 2019 IEEE 58th Conference on Decision and Control (CDC), 8230–8235, 2019. a
Heintz, C., Bailey, S. C., and Hoagg, J.: Formation Control of Fixed-Wing Unmanned Aircraft: Theory and Experiments, in: AIAA Scitech 2019 Forum, https://doi.org/10.2514/6.2019-1168, 2019. a
Jacob, J. D., Simpson, A., and Smith, S.: Design and Flight Testing of Inflatable Wings with Wing Warping, in: Aerospace Technology Conference and Exposition, SAE International, https://doi.org/10.4271/2005-01-3392, 2005. a
Jacob, J. D., Smith, S. W., Cadogan, D., and Scarborough, S.: Expanding the Small UAV Design Space with Inflatable Wings, in: Aerospace Technology Conference and Exposition, SAE International, https://doi.org/10.4271/2007-01-3911, 2007. a
Jacob, J. D., Chilson, P. B., Houston, A. L., and Smith, S. W.: Considerations for Atmospheric Measurements with Small Unmanned Aircraft Systems, Atmosphere, 9, 252, https://doi.org/10.3390/atmos9070252, 2018. a
Lenschow, D.: The measurement of air velocity and temperature using the NCAR Buffalo aircraft measuring system, National Center for Atmospheric Research, 1972. a
Lippay, Z. S. and Hoagg, J. B.: Leader-Following Formation Control in a Rotating Frame for Agents with Double-Integrator Dynamics: Generalized Stability Results and Experiments, in: 2019 IEEE 58th Conference on Decision and Control (CDC), 8236–8241, 2019. a
Mullen, J., Bailey, S. C., and Hoagg, J. B.: Filtered dynamic inversion for altitude control of fixed-wing unmanned air vehicles, Aerosp. Sci. Technol., 54, 241–252, https://doi.org/10.1016/j.ast.2016.04.013, 2016. a
Schuyler, T. J. and Guzman, M. I.: Unmanned Aerial Systems for Monitoring Trace Tropospheric Gases, Atmosphere, 8, 206, https://doi.org/10.3390/atmos8100206, 2017. a
Schuyler, T. J., Bailey, S. C. C., and Guzman, M. I.: Monitoring Tropospheric Gases with Small Unmanned Aerial Systems (sUAS) during the Second CLOUDMAP Flight Campaign, Atmosphere, 10, 434, https://doi.org/10.3390/atmos10080434, 2019a. a
Schuyler, T. J., Gohari, S. M. I., Pundsack, G., Berchoff, D., and Guzman, M. I.: Using a Balloon-Launched Unmanned Glider to Validate Real-Time WRF Modeling, Sensors, 19, 1914, https://doi.org/10.3390/s19081914, 2019b. a
Smith, S. W., Chilson, P. B., Houston, A. L., and Jacob, J. D.: Catalyzing Collaboration for Multi-Disciplinary UAS Development with a Flight Campaign Focussed on Meteorology and Atmospheric Physics, in: AIAA Information Systems-AIAA Infotech @ Aerospace, AIAA 2017-1156, AIAA, Grapevine, Texas, https://doi.org/10.2514/6.2017-1156, 2017. a
Thamann, M. A., Smith, S. W., Bailey, S. C., Doepke, E. B., and Ashcraft, S. W.: Modeling and flight testing of wing shaping for roll control of an unmanned aerial vehicle, Journal of Unmanned Vehicle Systems, 3, 192–204, https://doi.org/10.1139/juvs-2014-0024, 2015. a
Treaster, A. L. and Yocum, A. M.: The calibration and application of five-hole probes, Tech. rep., DTIC Document, 1978. a
Wellman, B. and Hoagg, J.: Sampled-Data Flocking with Application to Unmanned Rotorcraft, in: 2018 AIAA Guidance, Navigation, and Control Conference, https://doi.org/10.2514/6.2018-1856, 2018. a
Wildmann, N., Hofsäß, M., Weimer, F., Joos, A., and Bange, J.: MASC – a small Remotely Piloted Aircraft (RPA) for wind energy research, Adv. Sci. Res., 11, 55–61, https://doi.org/10.5194/asr-11-55-2014, 2014. a
Witte, B. M., Schlagenhauf, C., Mullen, J., Helvey, J. P., Thamann, M. A., and Bailey, S.: Fundamental Turbulence Measurement with Unmanned Aerial Vehicles (Invited), in: 8th AIAA Atmospheric and Space Environments Conference, AIAA 2016-3584, AIAA, Washington, DC, https://doi.org/10.2514/6.2016-3584, 2016. a
Witte, B. M., Singler, R. F., and Bailey, S. C.: Development of an Unmanned Aerial Vehicle for the Measurement of Turbulence in the Atmospheric Boundary Layer, Atmosphere, 8, 195, https://doi.org/10.3390/atmos8100195, 2017. a, b, c, d
- Abstract
- Introduction
- Description of the UASs and ground systems
- Description of measurement location(s), flight strategies and completed flights/sampling
- Data processing and quality control
- Overview of data
- Example profiles
- Data availability
- Conclusions
- Author contributions
- Competing interests
- Special issue statement
- Financial support
- Review statement
- References
- Abstract
- Introduction
- Description of the UASs and ground systems
- Description of measurement location(s), flight strategies and completed flights/sampling
- Data processing and quality control
- Overview of data
- Example profiles
- Data availability
- Conclusions
- Author contributions
- Competing interests
- Special issue statement
- Financial support
- Review statement
- References